Spatially Defined Gene Delivery into Native Cells with the Red Light-Controlled OptoAAV Technology
Maximilian Hörner, Maximilian Hörner, Wilfried Weber, Wilfried Weber
Abstract
The OptoAAV technology allows spatially defined delivery of transgenes into native target cells down to single-cell resolution by the illumination with cell-compatible and tissue-penetrating red light. The system is based on an adeno-associated viral (AAV) vector of serotype 2 with an engineered capsid (OptoAAV) and a photoreceptor-containing adapter protein mediating the interaction of the OptoAAV with the surface of the target cell in response to low doses of red and far-red light. In this article, we first provide detailed protocols for the production, purification, and analysis of the OptoAAV and the adapter protein. Afterward, we describe in detail the application of the OptoAAV system for the light-controlled transduction of human cells with global and patterned illumination. © 2022 The Authors. Current Protocols published by Wiley Periodicals LLC.
Basic Protocol 1 : Production, purification, and analysis of PhyB-DARPinEGFR adapter protein
Basic Protocol 2 : Production, purification, and analysis of OptoAAV
Basic Protocol 3 : Red light-controlled viral transduction with the OptoAAV system
Support Protocol : Spatially resolved transduction of two transgenes with the OptoAAV system
INTRODUCTION
Technologies that allow controlled transfer of genetic information into target cells play a key role in fundamental and applied genetic research. In this context, viral vectors are of particular interest due to their high efficiency for both cell lines and primary cells and their prolonged transgene expression (Wang et al., 2021). To externally control transgene delivery, light is the optimal stimulus as it can be easily applied with high spatiotemporal precision in a reversible manner. As existing technologies for light-controlled viral gene delivery were hampered by the need for cytotoxic ultraviolet (UV) light (Goater et al., 2000; Ito et al., 2004; Pandori & Sano, 2000; Pandori et al., 2002; Ulrich-Vinther et al., 2002; Wang et al., 2019), the required uptake of viral vectors to both target and off-target cells before optical control (Bonsted et al., 2006; Bonsted, Hogset, Hoover, & Berg, 2005; Goater et al., 2000; Gomez, Gerhardt, Judd, Tabor, & Suh, 2016; Hagihara et al., 2020; Hogset et al., 2002; Ito et al., 2004; Tahara et al., 2019; Ulrich-Vinther et al., 2002; Wang et al., 2019), or the requirement for genetic pre-engineering of the target cells to express the photoreceptor (Gomez et al., 2016), we recently published the OptoAAV technology that overcomes these limitations (Hörner et al., 2021). The OptoAAV system allows the spatially resolved delivery of transgenes into native target cells upon illumination with low-intensity red light and is discussed in more detail in the section Background Information. The OptoAAV technology is based on an engineered adeno-associated viral vector of serotype 2 (OptoAAV) and an adapter protein (e.g., PhyB-DARPinEGFR) mediating the interaction of the OptoAAV with the surface of the target cells in a light-dependent manner. In this article, we first describe the production, purification, and analysis of the PhyB-DARPinEGFR adapter protein (Basic Protocol 1) and of the OptoAAV (Basic Protocol 2). Afterward, we give a detailed protocol of how to employ PhyB-DARPinEGFR and OptoAAV for the transduction of the human epidermoid carcinoma cell line A-431 in a light-dependent manner (Basic Protocol 3). Finally, we describe the spatially resolved transduction of an A-431 cell culture with two different transgenes by patterned illumination using photomasks (Support Protocol).
CAUTION : OptoAAV is an engineered adeno-associated viral vector of serotype 2. Follow all appropriate guidelines and regulations for the use and handling of this viral vector.
Basic Protocol 1: PRODUCTION, PURIFICATION, AND ANALYSIS OF PhyB-DARPinEGFR ADAPTER PROTEIN
In this protocol, the production, purification, and analysis of the adapter protein PhyB-DARPinEGFR is described. This adapter protein targets the OptoAAV (see Basic Protocol 2) to cell lines overexpressing the human epidermal growth factor receptor (EGFR) in a light-dependent manner. The protocol is identical for adapter proteins comprising other designed ankyrin repeat proteins (DARPins) specific for EpCAM, Her2, or CD4 as described in the original publication (Hörner et al., 2021). PhyB-DARPinEGFR is produced in Escherichia coli together with the biosynthesis enzymes HO1 and PcyA for the chromophore phycocyanobilin (PCB) which are encoded on the same plasmid (Hörner et al., 2019). Afterward, PhyB-DARPinEGFR is purified via its hexahistidine tag by immobilized metal affinity chromatography (IMAC). Finally, the concentration of PhyB-DARPinEGFR is determined by Bradford assay and the protein purity and identity is analyzed by SDS-PAGE followed by Zn2+ staining of the chromophore PCB and Coomassie staining of proteins.
Materials
-
BL21 Star (DE3) One Shot chemically competent E. coli (Thermo Fisher Scientific, cat. no. C601003)
-
Plasmid pHJW156 (Hörner et al., 2021)
-
Lysogeny broth (LB) medium (Luria/Miller, prepare according to the manufacturer's instructions, Carl Roth, cat. no. X968.1)
-
500× streptomycin stock solution (50 mg/ml, prepare from PanReac AppliChem, cat. no. A1852, sterilize by passing through a 0.22-µm filter)
-
1 M IPTG stock solution (prepare from Carl Roth, cat. no. CN08.4, sterilize by passing through a 0.22-µm filter)
-
Lysis buffer (see recipe)
-
Liquid nitrogen
-
100 mM TCEP stock solution (see recipe)
-
Wash buffer (see recipe)
-
Elution buffer (see recipe)
-
25% ethanol (prepare from Carl Roth, cat. no. T171.4)
-
Protein buffer (see recipe)
-
0.04% (w/v) sodium azide solution (prepare from Carl Roth, cat. no. 4221.1)
-
1 mg/ml BSA solution (prepare from MilliporeSigma, cat. no. 05479)
-
Bradford assay reagent (Bio-Rad, cat. no. 5000006)
-
5× SDS loading buffer (see recipe)
-
Protein ladder (Thermo Fisher Scientific, cat. no. 26616)
-
SDS-PAGE gel, 12% (w/v) polyacrylamide, prepare according to manufacturer's instructions of the Mini-PROTEAN Tetra Cell SDS-PAGE system (Bio-Rad)
-
1 mM zinc acetate solution (prepare from MilliporeSigma, cat. no. 96459)
-
Coomassie solution (see recipe)
-
Destaining solution (see recipe)
-
Thermoshaker for 1.5-ml microcentrifuge tubes (Analytik Jena, Biometra TSC ThermoShaker)
-
50-ml conical centrifuge tubes (Corning, cat. no. 352070)
-
Heated and refrigerated shaking incubator for bacteria (Eppendorf, Innova 44R Shaker, orbit diameter: 2 in.)
-
500-ml and 2-L baffled glass flasks
-
Cuvettes (VWR, cat. no. 634-0676)
-
Photometer (GE Healthcare, GeneQuant 1300)
-
Centrifuge Avanti J-26 XP (Beckman Coulter) with JLA-8.1000 rotor (Beckman Coulter) and 1-L centrifuge beakers (Beckman Coulter) for harvesting of bacteria
-
Ultra-low temperature freezer (–80°C, Sanyo, MDF-U54V)
-
Water bath at 37°C (PEQLAB, WB-4MS)
-
High pressure homogenizer (SPX Flow Technology, APV-2000)
-
Centrifuge Avanti J-26 XP (Beckman Coulter) with JA-25.50 rotor (Beckman Coulter) and 50-ml centrifuge beakers (Beckman Coulter) for clarification of lysate
-
Fast protein liquid chromatography (FPLC) system (GE Healthcare, ÄKTAexplorer)
-
Ni-NTA Superflow column, 5 ml (Qiagen, cat. no. 30761)
-
Spin-X UF 20 centrifugal concentrators, 10 kDa MWCO, PES (Corning, cat. no. 431488)
-
Centrifuge for 15- and 50-ml conical centrifuge tubes (Eppendorf, Centrifuge 5804 with rotor FA-45-6-30)
-
Pierce dextran desalting columns, 5 kDa MWCO, 10 ml (Thermo Fisher Scientific, cat. no. 43233)
-
1.5-ml microcentrifuge tubes (Sarstedt, cat. no. 72.690.001)
-
Spin-X UF 6 centrifugal concentrators, 10 kDa MWCO, PES (Corning, cat. no. 431483)
-
Syringe, 5 ml (B. Braun, cat. no. 4617053V)
-
0.22-μm PES syringe filters (Carl Roth, cat. no. P668.1)
-
96-well U-bottom plates (Carl Roth, cat. no. 9291.1)
-
96-well F-bottom plates (Carl Roth, cat. no. 9293.1)
-
Multichannel pipets (200 and 20 µl)
-
Microplate reader (Tecan, Infinite M200 Pro)
-
SDS-PAGE system (Bio-Rad, Mini-PROTEAN Tetra Cell)
-
Ethidium bromide agarose gel imaging system with UV light (Intas, Gel iX Imager)
NOTE : Prepare all solutions with ultrapure water obtained by reverse osmosis.
Production of PhyB-DARPinEGFR in E. coli
Day 1: Transformation
1.Transform E. coli BL21 Star (DE3) with the PhyB-DARPinEGFR production plasmid pHJW156:
-
Thaw 1 aliquot of chemically competent BL21 Star (DE3) cells on ice and add 1 µl plasmid pHJW156 (10-500 ng/µl) to 50 µl cells.
-
Mix by gently tapping the tube and incubate on ice 20 min.
-
Incubate tube at 42°C in a heat block for 45 s and incubate tube immediately afterwards on ice for 2 min.
-
Add 500 µl LB medium and incubate tube in a thermoshaker at 37°C with agitation at 750 rpm for 1 hr.
-
Inoculate 10 ml LB medium supplemented with 100 µg/ml streptomycin in a 50-ml conical centrifuge tube with the transformed cells and incubate overnight at 37°C with agitation at 150 rpm.
Day 2: Inoculation of expression culture and induction of protein production
2.Inoculate 100 ml LB medium supplemented with 100 µg/ml streptomycin in a 500-ml baffled flask with the overnight culture and grow at 37°C with agitation at 150 rpm for 4 hr (pre-culture).
3.Inoculate each of four 2-L baffled flasks containing 1 L LB medium supplemented with 100 µg/ml streptomycin with 20 ml pre-culture and grow at 30°C with agitation at 150 rpm until an OD600 of 0.6 to 0.8 is reached (expression culture). Measure OD600 regularly using cuvettes and a photometer.
4.Induce protein production by the addition of 1 ml 1 M IPTG stock solution to each expression culture (final concentration: 1 mM) and incubate at 18°C with agitation at 150 rpm in the dark for 20 hr.
Day 3: Harvesting of expression culture
5.Harvest bacteria by centrifugation at 6,500 × g at 4°C for 10 min.
6.Discard supernatants and resuspend bacterial pellets in lysis buffer (30 ml lysis buffer per 1 L expression culture). Aliquot resuspended bacteria in 50-ml conical centrifuge tubes (40 ml per tube), snap freeze in liquid nitrogen, and store at −80°C.
Purification of PhyB-DARPinEGFR by IMAC
7.Thaw resuspended bacteria in a water bath at 37°C and transfer tubes after thawing immediately on ice.
8.Add TCEP to a final concentration of 0.5 mM from 100 mM TCEP stock solution.
9.Lyse bacteria by passing three times through a high-pressure homogenizer or French press at 1,000 to 1,500 bar. Cool lysate after each cycle on ice below 5°C.
10.Centrifuge lysate at 30,000 × g for 30 min at 4°C and transfer clarified supernatant into a new bottle.
11.Connect a Ni-NTA Superflow column [column volume (CV): 5 ml] to a FPLC system. For the purification process, use a flow rate of 5 ml/min. Detect absorbance at 280 nm. The purification should be performed at 4°C.
12.Equilibrate column with 25 ml (5 CV) of lysis buffer.
13.Load clarified supernatant on the column.
14.Wash column with 100 ml (20 CV) of wash buffer.
15.Elute protein with 25 ml (5 CV) of elution buffer and collect 2-ml fractions. Merge all turquoise-colored fractions containing PhyB-DARPinEGFR.
16.Concentrate eluted protein to ∼1 ml using an ultrafiltration centrifugal concentrator (10 kDa MWCO, PES membrane, 20 ml) at 6,000 × g and 20°C.
17.Equilibrate the 10-ml desalting column with 100 ml protein buffer.
18.Apply concentrated protein (∼1 ml) on the desalting column.
19.Add 1 ml protein buffer on the desalting column and discard flow-through.
20.Add 500 µl protein buffer and collect flow-through in a 1.5-ml microcentrifuge tube. Repeat this step until the colored protein is eluted from the column (usually within 5 ml).
21.Merge the six most intense turquoise-colored fractions (total volume: 3 ml) containing PhyB-DARPinEGFR.
22.Concentrate merged fractions to ∼1 ml using an ultrafiltration centrifugal concentrator (10 kDa MWCO, PES membrane, 6 ml) at 8,000 × g and 20°C.
23.Sterilize protein sample by passing through a 0.22-µm syringe filter.
24.Prepare 20-µl aliquots of the protein in 1.5-ml microcentrifuge tubes, snap freeze in liquid nitrogen, and store at −80°C.
Analysis of PhyB-DARPinEGFR
25.Thaw one aliquot of PhyB-DARPinEGFR in a heat block at 37°C and immediately transfer to ice upon thawing.
26.Determine protein concentration by Bradford assay in the 96-well plate format according to the manufacturer's instructions using BSA as a standard.
27.Analyze protein identity and purity by SDS-PAGE:
-
Mix 5 µl protein with 35 µl protein buffer and 10 µl 5× SDS loading buffer and incubate at 95°C for 5 min.
-
Separate 10 µl of sample and 5 µl of protein ladder on a 12% SDS-PAGE gel.
-
Incubate SDS-PAGE gel in a 1 mM zinc acetate solution for 15 min and visualize fluorescence of the PhyB-DARPinEGFR-bound chromophore PCB under UV light (312 nm) using a standard documentation system for ethidium-bromide-stained agarose gels.
-
Incubate the same agarose gel in Coomassie solution for 1 hr followed by incubation in destaining solution overnight.
Representative results of the SDS-PAGE analysis are depicted in Figure1.
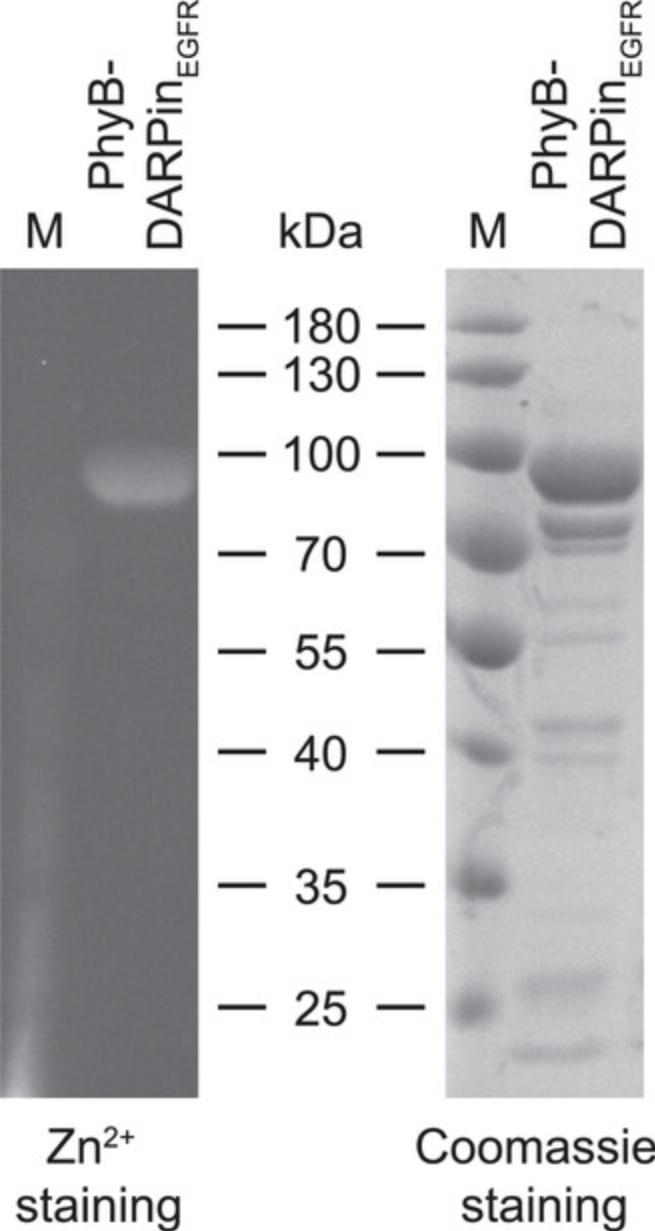
Basic Protocol 2: PRODUCTION, PURIFICATION, AND ANALYSIS OF OptoAAV
In this protocol, the production, purification, and analysis of OptoAAV is described. OptoAAV is an AAV vector of serotype 2 with a knockout of its natural tropism toward heparan sulfate proteoglycan (HSPG) that can transduce target cells in the presence of an adapter protein (see Basic Protocol 1) in response to the illumination with red light. OptoAAV is produced using the helper-free packaging system (Xiao, Li, & Samulski, 1998) in human embryonic kidney (HEK)-293T cells upon transfection of the required plasmids with polyethylenimine (PEI). Afterward, assembled OptoAAV capsids are purified by iodixanol gradient ultracentrifugation (Zolotukhin et al., 1999). Finally, the genomic titer of the purified OptoAAV is determined by quantitative real-time PCR (qPCR) and the composition of the viral capsids are analyzed by Western blotting against the viral capsid proteins VP1, VP2, and VP3.
Materials
-
HEK-293T cells (DSMZ, cat. no. ACC 635, RRID: CVCL 0063)
-
Dulbecco's modified Eagle's medium (DMEM, PAN Biotech, cat. no. P04-03550)
-
FBS (PAN Biotech, cat. no. P30-3602)
-
Penicillin/streptomycin solution (PAN Biotech, cat. no. P06-07100)
-
High-purity DNA of plasmid pHelper (Cell Biolabs, cat. no. VPK-402)
-
High-purity DNA of plasmid pRCVP2koA (gift from H. Büning, Hannover Medical School; Münch et al., 2013)
-
High-purity DNA of plasmid pMH303 (Hörner et al., 2021)
-
High-purity DNA of plasmid pCMVgfp (gift from D. Grimm, Heidelberg University)
-
Opti-MEM (Thermo Fisher Scientific, cat. no. 22600-134)
-
Polyethylenimine (PEI) solution (see recipe)
-
1× PBS (see recipe)
-
AAV lysis buffer (see recipe)
-
Liquid nitrogen
-
Benzonase nuclease, 25 U/µl (Merck, cat. no. 70664-3)
-
15% iodixanol solution (see recipe)
-
25% iodixanol solution (see recipe)
-
40% iodixanol solution (see recipe)
-
60% iodixanol solution (see recipe)
-
5× SDS loading buffer (see recipe)
-
Protein ladder (Thermo Fisher Scientific, cat. no. 26616)
-
SDS-PAGE gel, 12% (w/v) polyacrylamide, prepare according to manufacturer's instructions of the Mini-PROTEAN Tetra Cell SDS-PAGE system (Bio-Rad)
-
Polyvinylidene difluoride (PVDF) membrane (Merck Millipore, cat. no. IPVH00010)
-
Blocking buffer: 1× PBS (see recipe) supplemented with 3% (w/v) milk powder (Carl Roth, cat. no. T145.3)
-
Anti-AAV VP1/VP2/VP3 B1 antibody (Progen, cat. no. 65158)
-
PBS-T: 1× PBS (see recipe) supplemented with 0.05% (v/v) Tween 20 (Carl Roth, cat. no. 9127.2)
-
Anti-mouse horseradish peroxidase (HRP)-conjugated antibody (GE Healthcare, cat. no. NA931)
-
ECL Prime Western blotting detection reagent (GE Healthcare, cat. no. RPN2232)
-
ABsolute qPCR SYBR Green ROX mix (Thermo Fisher Scientific, cat. no. AB1163A)
-
Oligonucleotide CMV_fw (5′-TGCCCAGTACATGACCTTATGG-3′, 100 µM, custom synthesis by MilliporeSigma)
-
Oligonucleotide CMV_rv (5′-GAAATCCCCGTGAGTCAAACC-3′, 100 µM, custom synthesis by MilliporeSigma)
-
15-cm tissue culture plates (Corning, cat. no. 430599)
-
Laminar flow hood (Thermo Fisher Scientific, Safe 2020)
-
Cell culture incubator (Thermo Fisher Scientific, Heracell 240i)
-
50-ml conical centrifuge tubes (Corning, cat. no. 352070)
-
Vortexer (IKA, Cortex 3)
-
Cell lifter (Corning, cat. no. 3008)
-
Centrifuge with swing-out rotor for 50-ml conical centrifuge tubes (Eppendorf, Centrifuge 5804 with rotor S-4-72)
-
Water bath at 37°C (PEQLAB, WB-4MS)
-
Centrifuge for 15- and 50-ml conical centrifuge tubes (Eppendorf, Centrifuge 5804 with rotor FA-45-6-30)
-
Ultrasonic water bath (Elma, Elmasonic S 80 H)
-
15-ml conical centrifuge tubes (Greiner Bio-One, cat. no. 188271)
-
Ultracentrifugation tubes (Quick-Seal 13.5 ml, 16 × 76 mm, Beckman Coulter, cat. no. 342413)
-
Pasteur pipet (tip length at least 10 cm, Carl Roth, cat. no. 4522.1)
-
Cordless Tube Topper (Beckman Coulter, cat. no. 358313)
-
Ultracentrifuge (Beckman Coulter, Optima L-90 K with Type 70.1 Ti rotor)
-
Laboratory stand with clamp
-
Hollow needles, 0.9 × 40 mm (B. Braun, cat. no. 4657519)
-
Syringes, 3 ml (B. Braun, cat. no. 4617022V)
-
1.5-ml microcentrifuge tubes (Sarstedt, cat. no. 72.690.001)
-
Ultra-low temperature freezer (–80°C, Sanyo, MDF-U54V)
-
Thermoshaker for 1.5-ml microcentrifuge tubes (Analytik Jena, Biometra TSC ThermoShaker)
-
SDS-PAGE system (Bio-Rad, Mini-PROTEAN Tetra Cell)
-
Semi-dry electroblotting system (VWR, PerfectBlue)
-
Chemiluminescence imaging system (GE Healthcare, ImageQuant LAS 4000 Mini System)
-
384-well qPCR plate (Bio-Rad, cat. no. HSP3865)
-
384-well plate sealing film (Bio-Rad, cat. no. MSB1001)
-
qPCR thermocycler (Bio-Rad, CFX384)
Production of OptoAAV
Day 1: Cell seeding
1.Seed each of twenty 15-cm tissue culture plates with 8 × 106 HEK-293T cells in 20 ml complete DMEM (DMEM supplemented with 10%, v/v, FBS and 1%, v/v, penicillin/streptomycin solution). Incubate cells 24 hr at 37°C, 5% CO2 in a humidified atmosphere.
Day 2: Transfection with PEI
2.Dilute 470 µg plasmid pHelper, 295 µg plasmid pRCVP2koA, 250 µg plasmid pMH303, and 185 µg plasmid pCMVgfp in 30 ml Opti-MEM in a 50-ml conical centrifuge tube. Vortex and distribute solution equally in two 50-ml conical centrifuge tubes.
3.Dilute 4 ml PEI solution in 30 ml Opti-MEM in a 50-ml conical centrifuge tube. Vortex.
4.Add 17 ml PEI-Opti-MEM solution to each DNA-Opti-MEM solution and vortex immediately for 15 s.
5.Incubate both DNA-PEI-Opti-MEM mixes for 15 min at room temperature.
6.Add 4 ml of DNA-PEI-Opti-MEM mix dropwise (distributed over the whole plate) to each of the twenty, 15-cm tissue culture plates with HEK-293T cells. Gently move plate left and right and forward and backward to homogeneously distribute the transfection mix.
7.Incubate cells 6 hr at 37°C, 5% CO2 in a humidified atmosphere.
8.Aspirate medium of the plates and replace each with 20 ml complete DMEM.
9.Incubate cells for 72 hr at 37°C, 5% CO2 in a humidified atmosphere.
Day 5: Harvesting of OptoAAV
As OptoAAVs produced on ten plates will be purified by one iodixanol gradient (see below), the following harvesting protocol is described for ten plates. For harvesting of the twenty plates, perform the protocol twice.
10.Scrape cells from ten plates using a cell lifter without removing medium.
11.Resuspend cells and transfer them into 50-ml conical centrifuge tubes. Rinse plates with PBS (40 ml PBS for ten plates) and transfer resuspended cells into 50-ml conical centrifuge tubes.
12.Centrifuge cells at 400 × g for 10 min (swing-out rotor).
13.Discard supernatant and resuspend cells in 10 ml PBS per tube. Merge resuspended cells in one 50-ml conical centrifuge tube.
14.Centrifuge cells at 400 × g for 10 min (swing-out rotor).
15.Discard supernatant and resuspend cells in 5 ml AAV lysis buffer.
16.Lyse cells by five freeze-thaw cycles:
-
Freeze sample in liquid nitrogen for 5 min;
-
Thaw sample in a water bath at 37°C;
-
Once the sample is thawed, continue with step a. Repeat this procedure four times.
Once the cell suspension is frozen in liquid nitrogen, it can be stored at −80°C.
Purification of OptoAAV by iodixanol gradient ultracentrifugation
NOTE : The following section of the protocol describes the preparation of one iodixanol gradient that allows purification of OptoAAVs produced on ten plates. For the purification from twenty plates, prepare two iodixanol gradients. Dependent on the number of parallel purifications, an additional iodixanol gradient loaded with AAV lysis buffer instead of the AAV suspension may have to be prepared for balancing purposes.
17.Sonicate sample containing the lysed cells of ten plates for 80 s in an ultrasonic water bath.
18.Add 10 µl benzonase (final concentration: 50 U/ml) and incubate at 37°C in a water bath for 1 hr. Briefly vortex sample every 10 min.
19.Centrifuge sample at 4,000 × g for 15 min at 4°C.
20.Transfer supernatant into a new 15-ml conical centrifuge tube. Fill up sample to 7 ml with AAV lysis buffer and repeat centrifugation. Transfer supernatant in a new 15-ml conical centrifuge tube.
21.Prepare iodixanol gradient:
-
Put a glass Pasteur pipet into an ultracentrifugation tube. The thin end of the Pasteur pipet must touch the bottom of the tube.
-
Pipet AAV suspension (∼7 ml) into the Pasteur pipet. The AAV suspension will be transferred through the Pasteur pipet to the bottom of the ultracentrifugation tube.
-
Pipet 1.5 ml of the 15% iodixanol solution into the Pasteur pipet. The iodixanol solution will displace the AAV suspension upward and will be the lowest phase.
-
Pipet 1.5 ml of the 25% iodixanol solution into the Pasteur pipet. This solution will displace all other phases upward and will be the lowest phase.
-
Pipet 1.5 ml of the 40% iodixanol solution into the Pasteur pipet. This solution will displace all other phases upward and will be the lowest phase.
-
Pipet 1.5 ml of the 60% iodixanol solution into the Pasteur pipet. This solution will displace all other phases upward and will be the lowest phase. The AAV suspension should now touch the upper border of the ultracentrifugation tube.
-
Close the upper opening of the Pasteur pipet with a finger and carefully remove the pipet.
-
Fill up the ultracentrifugation tube with AAV lysis buffer using a thin gel loading tip until the solution reaches the middle of the tube stem. Make sure to remove all air bubbles with the gel loading tip.
If the liquid flow in the Pasteur pipet stops, gently rotate the pipet. The ultracentrifugation tube should not contain any air bubbles. If air bubbles are trapped within the tube, gently tap the tube to move the air bubbles upward. Carefully move the tube only vertically to avoid destruction of the step gradient.
22.Seal the ultracentrifugation tube according to the manufacturer's instructions. Briefly, place a seal former on the tube stem. Pre-heat the Tube Topper for 5 s and apply the tip of the Tube Topper vertically to the seal former. Within a few seconds, the seal former will move down the tube stem until it reaches the tube shoulder. Immediately remove the Tube Topper and place the heat sink on the seal former for 15 s. Remove the seal former from the sealed tube.
23.Place two ultracentrifugation tubes into the type 70.1 Ti rotor and place the Quick-Seal tube spacers on top.
24.Centrifuge at 50,000 rpm at 4°C for 2 hr.
25.Remove ultracentrifugation tubes from the rotor and harvest the 40% iodixanol fraction containing purified OptoAAV:
-
Fix ultracentrifugation tube with the clamp of a lab stand.
-
Puncture upper phase at the top of the tube with a hollow needle (0.9 × 40 mm) to allow air to flow in.
-
Attach a hollow needle (0.9 × 40 mm) to a 3-ml syringe and puncture the ultracentrifugation in the 60% iodixanol phase, ∼1 mm below the border to the 40% iodixanol phase. Face the bevel of the needle upward at the interface between the 60% and 40% iodixanol phase and collect ∼1.2 ml of the 40% iodixanol phase. Harvesting of the OptoAAV from the iodixanol gradient is illustrated in Figure2.
The OptoAAV will collect mainly in the 40% iodixanol phase and at the interface between the 60% and 40% iodixanol phase. Avoid collecting the cellular protein-rich band near the 40% to 25% iodixanol density junction.
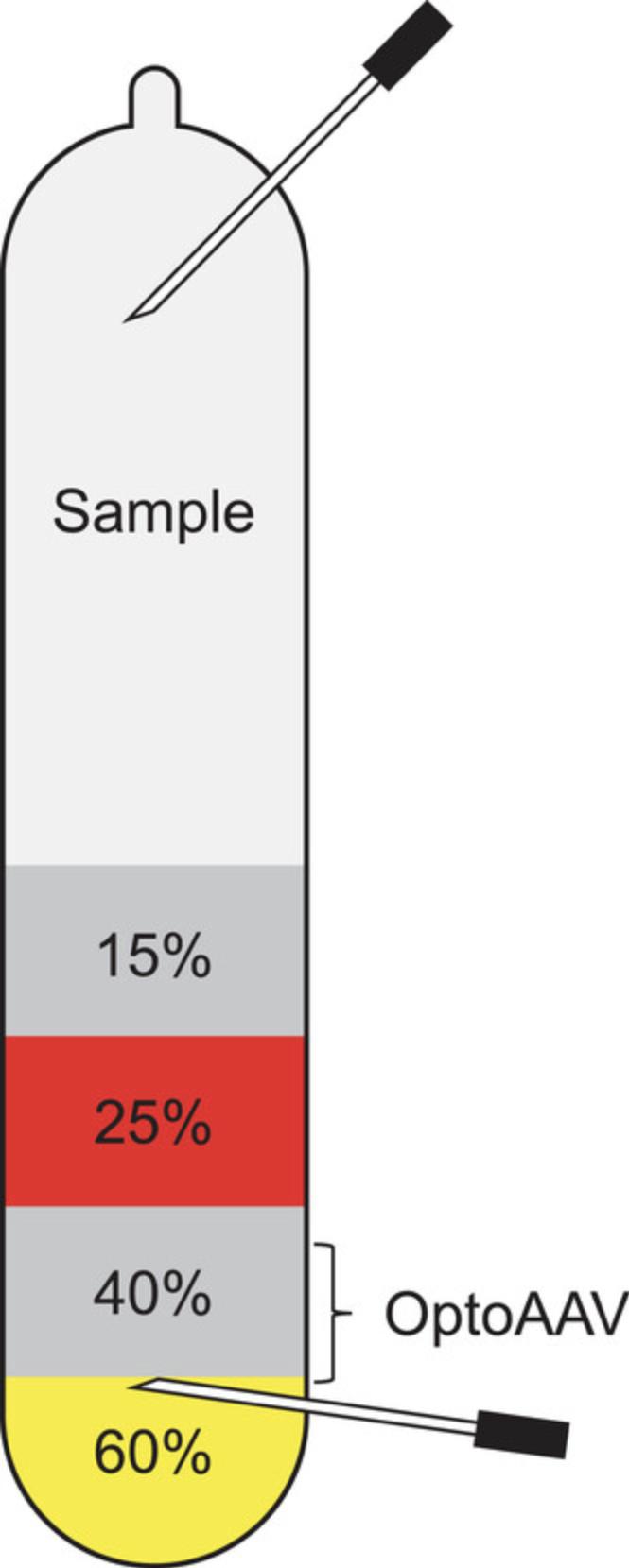
26.Transfer extracted OptoAAV from the 40% iodixanol phase into a 1.5-ml microcentrifuge tube. Carefully mix and prepare 50-µl aliquots in 1.5-ml microcentrifugation tubes, snap freeze in liquid nitrogen, and store at −80°C.
Analysis of purified OptoAAV
27.Thaw one aliquot of purified OptoAAV in a heat block at 37°C and immediately transfer to ice upon thawing.
28.Analyze capsid proteins by SDS-PAGE and Western blotting:
-
Mix 40 µl purified OptoAAV with 10 µl of 5× SDS loading buffer.
-
Incubate at 95°C for 5 min.
-
Separate 10 µl of sample and 5 µl of protein ladder on a 12% SDS-PAGE gel.
-
Transfer proteins onto a PVDF membrane by electroblotting.
-
Block membrane by incubation in blocking buffer for 1 hr.
-
Incubate membrane with B1 antibody, diluted 1:100 in blocking buffer at 4°C overnight.
-
Wash membrane three times with PBS-T.
-
Incubate membrane with secondary anti-mouse HRP-conjugated antibody, diluted 1:2,000 in blocking buffer for 1 hr.
-
Wash membrane three times with PBS-T.
-
Add Western blotting detection reagent and image chemiluminescence.
Representative results are depicted in Figure3.
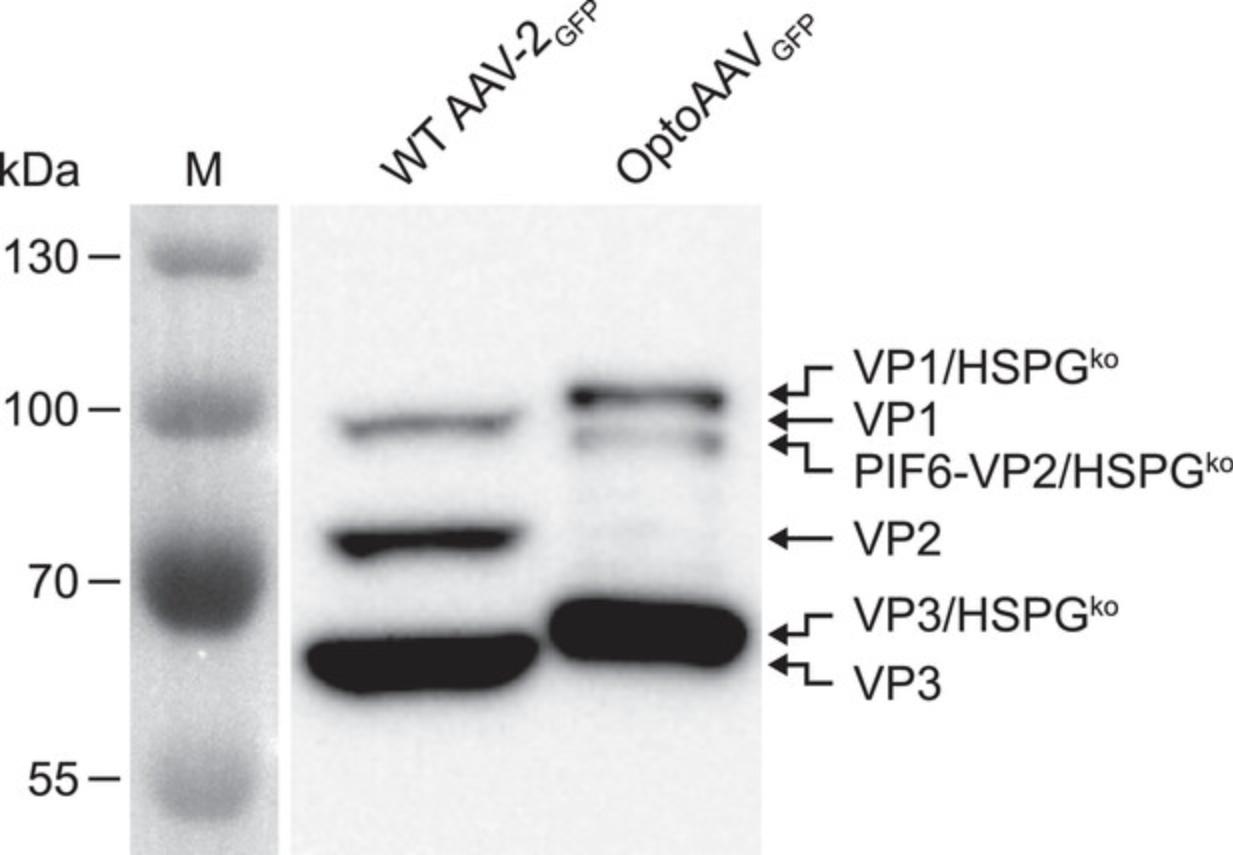
29.Determination of genomic titer by qPCR:
-
Prepare dilution series of the purified OptoAAV in water (start dilution: 1:1,000, prepare 1:5 dilution series with three dilutions).
-
Prepare dilution series of the vector plasmid pCMVgfp in water (start concentration: 2 × 108molecules/ml, prepare 1:10 dilution series with five dilutions, plasmid size: 4.6 kbp).
-
Prepare master mix for qPCR reaction (amounts for one well/reaction):10 µl ABsolute qPCR SYBR Green ROX mix;0.15 µl CMV_fw primer (100 µM);0.15 µl CMV_rv primer (100 µM);4.7 µl water.
-
Add 15 µl of master mix per well of the 384-well plate.
-
Add 5 µl of sample to each well.
-
Seal plate with foil and run qPCR with the following program:95°C, 10 min;95°C, 15 s;67°C, 5 s;72°C, 10 s;Repeat steps ii to iv thirty-nine times.
-
Determine Cq values with the qPCR machine software. Calculate the genomic OptoAAV titer (vg/ml) based on the standard curve and the OptoAAV dilutions.
We obtained an average genomic titer of 1.6 × 1011vector genomes (vg) for OptoAAV produced in ten 15-cm plates.
Basic Protocol 3: RED LIGHT-CONTROLLED VIRAL TRANSDUCTION WITH THE OptoAAV SYSTEM
In this protocol, the light-controlled viral transduction of the human epidermoid carcinoma cell line A-431 using the OptoAAV technology is described. The OptoAAV system requires the purified adapter protein PhyB-DARPinEGFR (Basic Protocol 1) and the purified OptoAAV (Basic Protocol 2). The transduction efficiency of the OptoAAV system and the impact of the two individual components (PhyB-DARPinEGFR and OptoAAV) on the cells are determined. Viral transduction is analyzed by measuring expression of the OptoAAV model transgene gfp by flow cytometry. Spatially resolved transduction is described in the Support Protocol.
Materials
-
A-431 cells (DSMZ, cat. no. ACC 91, RRID: CVCL 0037)
-
Dulbecco's modified Eagle's medium (DMEM, PAN Biotech, cat. no. P04-03550)
-
FBS (PAN Biotech, cat. no. P30-3602)
-
Penicillin/streptomycin solution (PAN Biotech, cat. no. P06-07100)
-
OptoAAVGFP (production described in Basic Protocol 2)
-
PhyB-DARPinEGFR (production described in Basic Protocol 1)
-
Protein buffer (see recipe)
-
PBS-BSA: 1× PBS (see recipe) supplemented with 1% (w/v) BSA (MilliporeSigma, cat. no. 05479)
-
1× PBS (see recipe)
-
Trypsin/EDTA solution (PAN Biotech, cat. no. P10-023500)
-
PBS-FBS: 1× PBS (see recipe) supplemented with 5% (v/v) FBS
-
96-well tissue culture plates (Corning, cat. no. 3599)
-
Laminar flow hood (Thermo Fisher Scientific, Safe 2020) in a room that can be completely shaded
-
Cell culture incubator (Thermo Fisher Scientific, Heracell 240i)
-
Thermoshaker for 1.5-ml microcentrifuge tubes (Analytik Jena, Biometra TSC ThermoShaker)
-
1.5-ml microcentrifuge tubes (Sarstedt, cat. no. 72.690.001)
-
Microcontroller-regulated light-emitting diode (LED) panels with 660 nm (Opulent Americas, cat. no. LST1-01F06-PRD1-00) and 740 nm (LED Engin, cat. no. LZ4-00R308) LEDs (similar LED panels are described in Müller, Zurbriggen, & Weber, 2014; alternatively, OptoPlate-96 can be used, see Bugaj & Lim, 2019; Thomas, Hörner, & Weber, 2020)
-
Fiber-optic spectrometer to measure light intensities (Avantes, AvaSpec-ULS2048)
-
Light-emitting diode (LED) strip with green LEDs (Osram, Deco Flex LED strips)
-
Multichannel pipet (200 µl)
-
96-well plate for flow cytometer (Greiner Bio-One, cat. no. 650161)
-
Flow cytometer with GFP channel (Thermo Fisher Scientific, Attune NxT)
-
Flow cytometry data analysis software (Becton, Dickinson and Company, FlowJo)
Day 1: Cell seeding
1.Seed each of twelve wells of two 96-well tissue culture plates with 5,000 A-431 cells in 100 µl complete DMEM (DMEM supplemented with 10%, v/v, FBS and 1%, v/v, penicillin/streptomycin solution). Incubate cells 24 hr at 37°C, 5% CO2 in a humidified atmosphere.
Day 2: Light-controlled viral transduction
2.Thaw the required number of aliquots of purified OptoAAVGFP in a heat block at 37°C and immediately transfer to ice upon thawing.
3.Incubate the OptoAAVGFP aliquots at 62.5°C in a heat block for 10 min and transfer afterwards immediately to ice.
4.Thaw one aliquot of PhyB-DARPinEGFR in a heat block at 37°C and immediately transfer to ice upon thawing.
5.Dilute PhyB-DARPinEGFR 1:10 with protein buffer. Use the diluted protein in the next step.
6.Prepare the following samples in 1.5-ml microcentrifuge tubes:
-
Sample 1: 650 µl PBS-BSA;
-
Sample 2: 6.6 × 109vg OptoAAVGFPin PBS-BSA (total volume: 650 µl);
-
Sample 3: 50 nM PhyB-DARPinEGFRin PBS-BSA (total volume: 650 µl);
-
Sample 4: 6.6 × 109vg OptoAAVGFPand 50 nM PhyB-DARPinEGFRin PBS-BSA (total volume: 650 µl).
Assuming an OptoAAVGFP titer of 1.7 × 1011 vg/ml and a PhyB-DARPinEGFR concentration of 1.2 mg/ml (equals 13.4 µM, molecular weight: 89.4 kDa), this corresponds to:
- e.Sample 1: 650 µl PBS-BSA;
- f.Sample 2: 39 µl OptoAAVGFP, 611 µl PBS-BSA;
- g.Sample 3: 2.4 µl PhyB-DARPinEGFR, 648 µl PBS-BSA;
- h.
Sample 4: 39 µl OptoAAVGFP, 2.4 µl PhyB-DARPinEGFR, 609 µl PBS-BSA.
Assuming that the number of A-431 cells increased by 50% after seeding, this corresponds to a multiplicity of infection (MOI) of 1.4 × 105.
7.Illuminate the four samples 5 min with 740 nm light (20 µmol m-2 s-1).
8.In the meantime, aspirate medium of the two 96-well plates and add 100 µl PBS.
9.Switch off room light and switch on dim green light. Aspirate PBS and add 100 µl of each sample to each of three wells of both plates.
10.Illuminate one plate with pulsed 660 nm light (5 min ON, 55 min OFF; 20 µmol m-2 s-1) and the other plate with continuous 740 nm light (20 µmol m-2 s-1) for 2 hr at 37°C, 5% CO2 in a humidified atmosphere.
11.Work again only under dim green light. Aspirate samples from the wells and add 100 µl complete DMEM per well. Continue with the previous illumination for 46 hr at 37°C, 5% CO2 in a humidified atmosphere.
Day 4: Analysis of light-controlled viral transduction by flow cytometry
The following steps can be performed under normal room light.
12.Aspirate medium and add 100 µl PBS per well.
13.Aspirate PBS and add 50 µl trypsin/EDTA solution per well. Incubate 15 min at 37°C.
14.Add 200 µl ice-cold PBS-FBS to each well. Resuspend seven times and transfer 200 µl of each well into the 96-well plate for flow cytometry. Cover the 96-well plate with aluminum foil and store on ice until the measurement.
15.Analyze GFP fluorescence of the cells by flow cytometry.
16.Evaluate flow cytometry data. Briefly, gate for living cells based on FSC-A and SSC-A signals and exclude doublets based on the FSC-A and FSC-H signal. Exclude autofluorescent cells based on their fluorescence in an unused channel and finally gate for GFP-negative and GFP-positive cells based on the blank control.
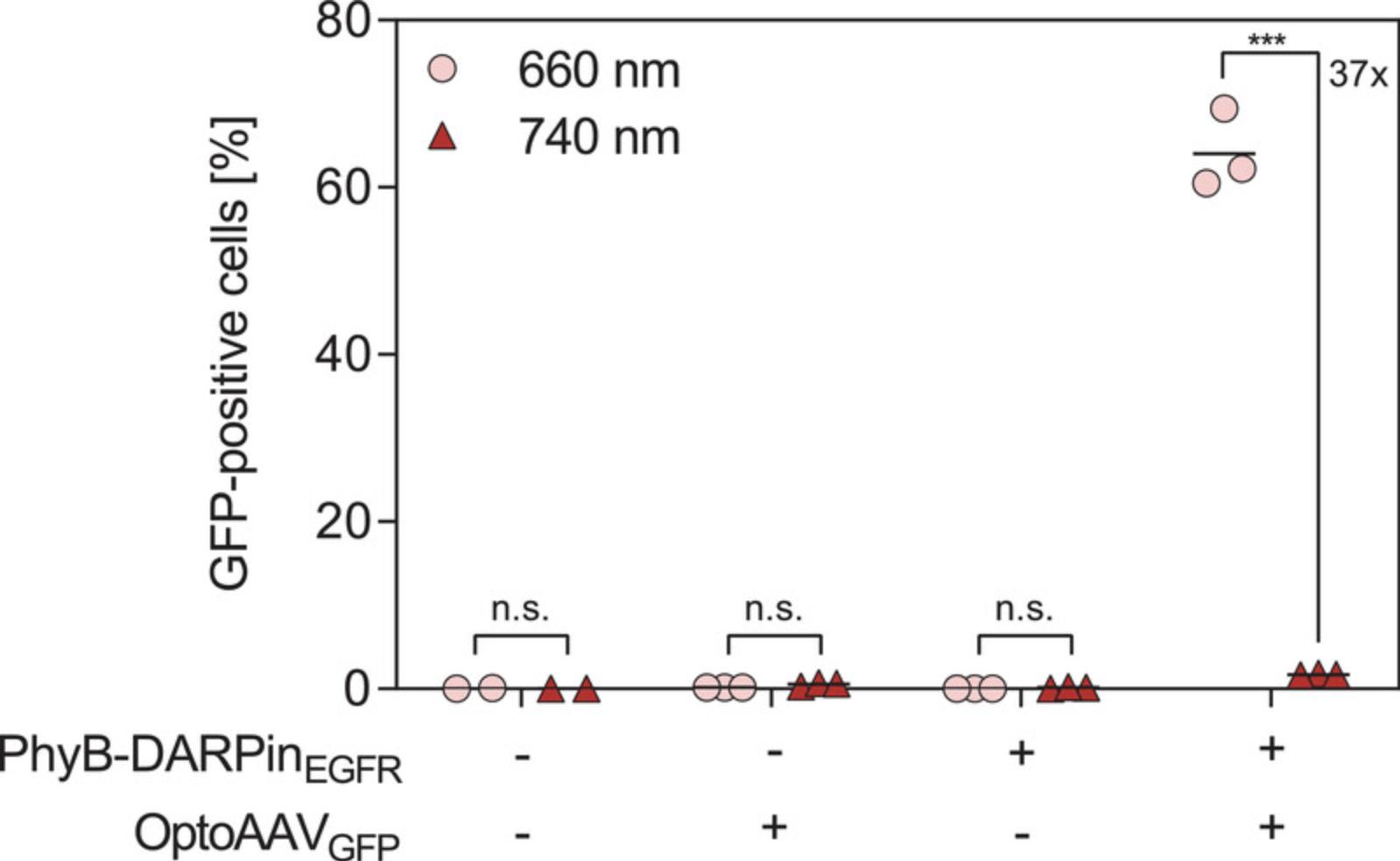
Support Protocol: SPATIALLY RESOLVED TRANSDUCTION OF TWO TRANSGENES WITH THE OptoAAV SYSTEM
This support protocol is an extension of Basic Protocol 3 and describes the spatially resolved transduction of different cells in the same culture with two different transgenes (gfp and mScarlet). The protocol requires an additional OptoAAV (encoding the red fluorescent protein mScarlet) that is produced according to Basic Protocol 2 employing the transfer plasmid pCMVmScarlet. Spatial illumination is achieved using two photomasks (photomask 1 for OptoAAVGFP and photomask 2 for OptoAAVmScarlet) and transduction is visualized by confocal microscopy.
Additional Materials (see also Basic Protocol 3)
-
OptoAAVmScarlet: Produced as described in Basic Protocol 2 but using the transfer plasmid pCMVmScarlet (gift from D. Grimm, Heidelberg University)
-
Paraformaldehyde (PFA) solution (see recipe)
-
DAPI solution: 1 µg/ml DAPI (MilliporeSigma, cat. no. D9542-5MG) in 1× PBS (see recipe)
-
µ-Slide four-well chambered coverslip (ibidi, cat. no. 80426)
-
Photomask 1 and 2 (laser photoplot films, 4,000 dpi, JD Photo Data) attached on glass plates
-
Fluorescence microscope (Zeiss LSM 880 laser scanning confocal microscope)
Day 1: Seeding of cells
1.Seed 1 × 105 A-431 cells in 750 µl complete DMEM in one well of a µ-slide four-well chambered coverslip. Incubate cells 24 hr at 37°C, 5% CO2 in a humidified atmosphere.
Day 2: Spatially resolved viral transduction
2.Dilute PhyB-DARPinEGFR and expose OptoAAVGFP and OptoAAVmScarlet to limited heat shock as described in steps 2-5 of Basic Protocol 3.
3.Prepare PhyB-DARPinEGFR solution: 50 nM PhyB-DARPinEGFR in PBS-FBS (total volume: 750 µl).
4.Prepare OptoAAVGFP solution: 2.3 × 109 vg OptoAAVGFP in PBS-FBS (total volume: 750 µl); corresponds to an MOI of 1.5 × 104.
5.Wash µ-slide well once with 750 µl PBS.
6.Add 750 µl PhyB-DARPinEGFR solution to the well. Incubate 10 min under 740 nm light (20 µmol m-2 s-1).
7.Switch off room light and switch on dim green light. Wash well once with 750 µl PBS.
8.Add 750 µl OptoAAVGFP solution to the well. Illuminate the well spatially resolved with 660 nm light:
-
Place µ-slide on photomask 1 that is attached on a glass plate.
-
Illuminate from below through the photomask with 660 nm light (20 µmol m-2s-1) for 9 s.
9.Incubate 2 hr at 37°C, 5% CO2 in a humidified atmosphere in the dark (wrap the µ-slide in aluminum foil).
10.Switch on room light: Prepare new PhyB-DARPinEGFR solution: 50 nM PhyB-DARPinEGFR in PBS-FBS (total volume: 750 µl).
11.Prepare OptoAAVmScarlet solution: 2.6 × 109 vg OptoAAVmScarlet in PBS-FBS (total volume: 750 µl); corresponds to an MOI of 1.7 × 104.
12.Switch off room light and switch on dim green light. Repeat steps 5-9 using OptoAAVmScarlet solution and photomask 2.
13.Switch off room light and switch on dim green light. Wash well once with 750 µl PBS.
14.Add 750 µl complete DMEM. Incubate 19.5 hr under 740 nm light (20 µmol m-2 s-1) at 37°C, 5% CO2 in a humidified atmosphere.
Day 3: Reduction of temperature
15.Reduce temperature of the cell culture incubator from 37°C to 30°C. Incubate for 24 hr.
Day 4: Fixation of cells and analysis by fluorescence microscopy
16.Wash µ-slide well once with 750 µl PBS.
17.Add 750 µl PFA solution and incubate 20 min.
18.Wash well once with 750 µl PBS.
19.Add 750 µl DAPI solution and incubate 20 min.
20.Wash well once with 750 µl PBS.
21.Analyze spatially resolved transduction by imaging DAPI (cell nuclei), GFP, and mScarlet fluorescence of the cells by confocal microscopy.
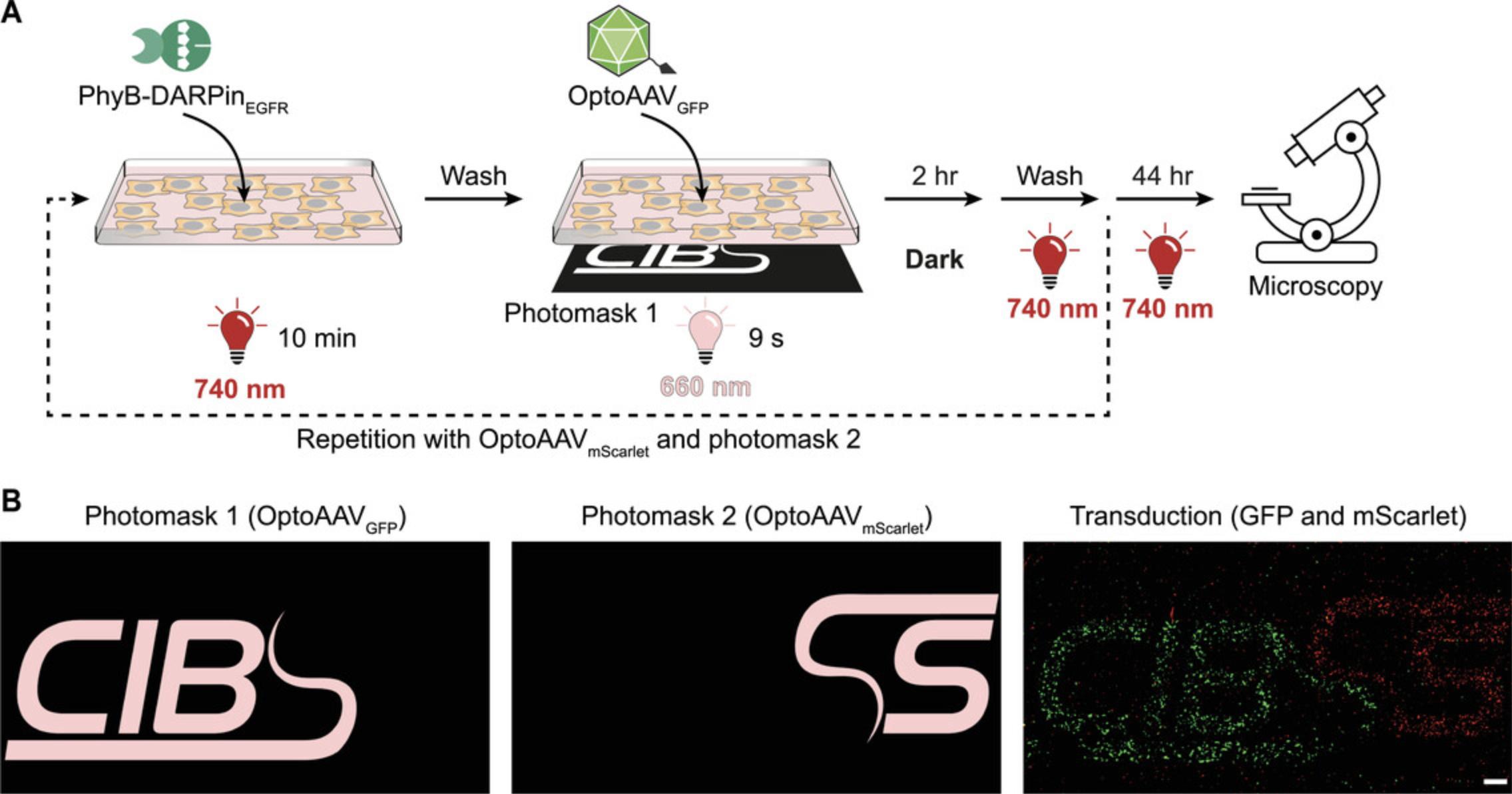
REAGENTS AND SOLUTIONS
Prepare all solutions with ultrapure water obtained by reverse osmosis.
AAV lysis buffer
- 50 mM Tris·HCl, pH 8.5
- 150 mM NaCl
- For the preparation of 1 L AAV lysis buffer, add 6.1 g Tris and 8.8 g NaCl. Fill up with water to 0.9 L. Adjust pH with HCl to 8.5. Fill up with water to 1 L. Autoclave.
- Store at room temperature for up to 1 year (Carl Roth, cat. no. 3862.2).
Coomassie solution
- 10% (v/v) acetic acid
- 25% (v/v) 2-propanol
- 0.1% (w/v) Coomassie brilliant blue R 250 (Carl Roth, cat. no. 3862.2)
- For the preparation of 1 L Coomassie solution, add 100 ml acetic acid, 250 ml 2-propanol, and 1 g Coomassie brilliant blue R 250. Fill up to 1 L with water.
- Store at room temperature for up to 1 year.
Destaining solution
- 10% (v/v) acetic acid
- 20% (v/v) ethanol
- For the preparation of 1 L destaining solution, add 100 ml acetic acid and 200 ml ethanol. Fill up to 1 L with water.
- Store at room temperature for up to 1 year.
Elution buffer
- 50 mM NaH2PO4·2H2O
- 300 mM NaCl
- 250 mM imidazole
- 0.5 mM TCEP (add directly before use)
- For the preparation of 1 L elution buffer, add 7.8 g NaH2PO4·2H2O, 17.5 g NaCl, and 17.0 g imidazole. Fill up with water to 0.9 L. Adjust pH with NaOH to 8.0. Fill up with water to 1 L.
- The buffer without TCEP can be stored at 4°C for up to 1 year.
- Directly before use, add 0.5 mM TCEP from 100 mM TCEP stock solution (see recipe). Do not store the buffer with TCEP.
Iodixanol solution, 15%
- 6 ml of 60% iodixanol (OptiPrep density gradient medium, MilliporeSigma, cat. no. D1556-250ML)
- 18 ml of PBS-MK-N (see recipe)
- Store at 4°C for up to 1 year.
Iodixanol solution, 25%
- 10 ml of 60% iodixanol (MilliporeSigma, cat. no. D1556-250ML)
- 14 ml of PBS-MK (see recipe)
- 60 µl of 0.5% (w/v) phenol red solution (MilliporeSigma, cat. no. P0290-100ML)
- Store at 4°C for up to 1 year.
- The solution is red.
Iodixanol solution, 40%
- 15 ml of 60% iodixanol (MilliporeSigma, cat. no. D1556-250ML)
- 7.5 ml of PBS-MK (see recipe)
- Store at 4°C for up to 1 year.
Iodixanol solution, 60%
- 25 ml of 60% iodixanol (MilliporeSigma, cat. no. D1556-250ML)
- 62.5 µl of 0.5% (w/v) phenol red solution
- Store at 4°C for up to 1 year.
- The solution is yellow.
Lysis buffer
- 50 mM NaH2PO4·2H2O
- 300 mM NaCl
- 10 mM imidazole
- For the preparation of 1 L lysis buffer, add 7.8 g NaH2PO4·2H2O, 17.5 g NaCl, and 0.68 g imidazole. Fill up with water to 0.9 L. Adjust pH with NaOH to 8.0. Fill up with water to 1 L.
- Store at 4°C for up to 1 year.
Paraformaldehyde (PFA) solution
- 4% (w/v) paraformaldehyde (PFA) in 1× PBS
- For the preparation of 1 L PFA solution, add 40 g PFA to 100 ml 10× PBS (see recipe) and 800 ml water. Heat to ∼50°C and mix using a magnetic stirring bar. Upon dissolution of PFA, fill up to 1 L with water.
- Prepare PFA solution in a fume hood.
- Store in aliquots at −20°C for up to 1 year.
PBS, 1×
- 100 ml 10× PBS (see recipe)
- 900 ml water
- Autoclave.
- Store at room temperature for up to at least 1 year.
PBS, 10×
- 26.7 mM KCl
- 14.7 mM KH2PO4
- 80.6 mM Na2HPO4·2H2O
- 1.37 M NaCl
- For the preparation of 1 L 10× PBS, add 2.0 g KCl, 2.0 g KH2PO4, 14.3 g Na2HPO4·2H2O, and 80.1 g NaCl. Fill up to 1 L with water. Autoclave.
- Store at room temperature for up to 1 year.
PBS-MK
- 1× PBS
- 1 mM MgCl2
- 2.5 mM KCl
- For the preparation of 100 ml PBS-MK, add 10 ml of 10× PBS, 100 µl of 1 M MgCl2, and 100 µl of 2.5 M KCl. Fill up to 100 ml with water. Sterilize by passing through a 0.22-µm filter.
- Store at room temperature for up to 1 year.
PBS-MK-N
- 1× PBS
- 1 mM MgCl2
- 2.5 mM KCl
- 1 M NaCl
- For the preparation of 100 ml PBS-MK-N, add 10 ml of 10× PBS, 100 µl of 1 M MgCl2, 100 µl of 2.5 M KCl, and 20 ml of 5 M NaCl. Fill up to 100 ml with water. Sterilize by passing through a 0.22-µm filter.
- Store at room temperature for up to 1 year.
Polyethylenimine (PEI) solution
- 1 mg/ml linear polyethylenimine (PEI; MW 25,000) in water (pH 7.0)
- For the preparation of PEI solution, add 170 ml water to 200 mg PEI (Polysciences, cat. no. 23966-1) and mix with a magnetic stirring bar. Add HCl until all PEI is dissolved and the pH is stable at 7.0. Fill up to 200 ml with water and check pH again. Sterilize the solution by passing through a 0.22-µm filter.
- Store in aliquots at −80°C for up to 1 year.
Use thawed aliquots only on the same day. Avoid repeated freeze-thaw cycles.
Protein buffer
- 1× PBS
- 0.5 mM TCEP
- For the preparation of 100 ml protein buffer, add 10 ml of 10× PBS and 500 µl of 100 mM TCEP stock solution (see recipe). Fill up to 100 ml with water.
- Do not store and always prepare fresh before use.
SDS loading buffer, 5×
- 0.31 M Tris·HCl (from 1 M Tris·HCl stock solution, pH 6.8)
- 50% (v/v) glycerol
- 10% (w/v) SDS
- 12.5% (v/v) 2-mercaptoethanol
- 0.05% (w/v) bromophenol blue sodium salt
- For the preparation of 200 ml 5× SDS loading buffer, dissolve 20 g SDS in 62.6 ml of 1 M Tris·HCl solution, pH 6.8. Add 100 ml glycerol, 100 mg bromophenol blue sodium salt, and 25 ml 2-mercaptoethanol. Fill up to 200 ml with water.
- Store in aliquots at −20°C for up to 1 year.
TCEP stock solution, 100 mM
- Freshly prepare 0.5 M NaHCO3 in water. Adjust pH to 9.0 with NaOH.
- Weigh 2.87 g TCEP hydrochloride and fill up with 0.5 M NaHCO3 (pH 9.0) solution to 100 ml. Adjust pH to 8.0 with NaOH and/or HCl and sterilize solution by passing through a 0.22-µm filter.
- Store in single-use aliquots at −20°C for up to 1 year.
Wash buffer
- 50 mM NaH2PO4·2H2O
- 300 mM NaCl
- 20 mM imidazole
- 0.5 mM TCEP (add directly before use)
- For the preparation of 1 L wash buffer, add 7.8 g NaH2PO4·2H2O, 17.5 g NaCl, and 1.4 g imidazole. Fill up with water to 0.9 L. Adjust pH with NaOH to 8.0. Fill up with water to 1 L.
- The buffer without TCEP can be stored at 4°C for up to 1 year.
- Directly before use, add 0.5 mM TCEP from 100 mM TCEP stock solution (see recipe). Do not store the buffer with TCEP.
COMMENTARY
Background Information
The OptoAAV technology allows the spatially resolved delivery of transgenes into native target cells down to single-cell resolution by the illumination with low-intensity red and far-red light (Hörner et al., 2021). The system is based on an engineered AAV vector of serotype 2 (OptoAAV) and an adapter protein. The viral capsid of the OptoAAV contains two modifications. First, the mutations R585A and R588A in the three viral capsid proteins ablate the binding of AAV2 to its primary receptor HSPG. Second, the capsid contains the phytochrome interacting factor 6 (PIF6) fused N-terminally to the capsid protein VP2. The adapter protein comprises phytochrome B (PhyB) fused to a DARPin that binds specifically to a selected cell surface protein. Illumination of PhyB with red (∼660 nm) light induces its interaction with PIF6 thereby recruiting the OptoAAV to the cell surface resulting in transduction of the target cell (Fig. 6). In contrast, illumination of PhyB with far-red (∼740 nm) light dissociates the interaction between PhyB and PIF6 and thus prevents transduction.
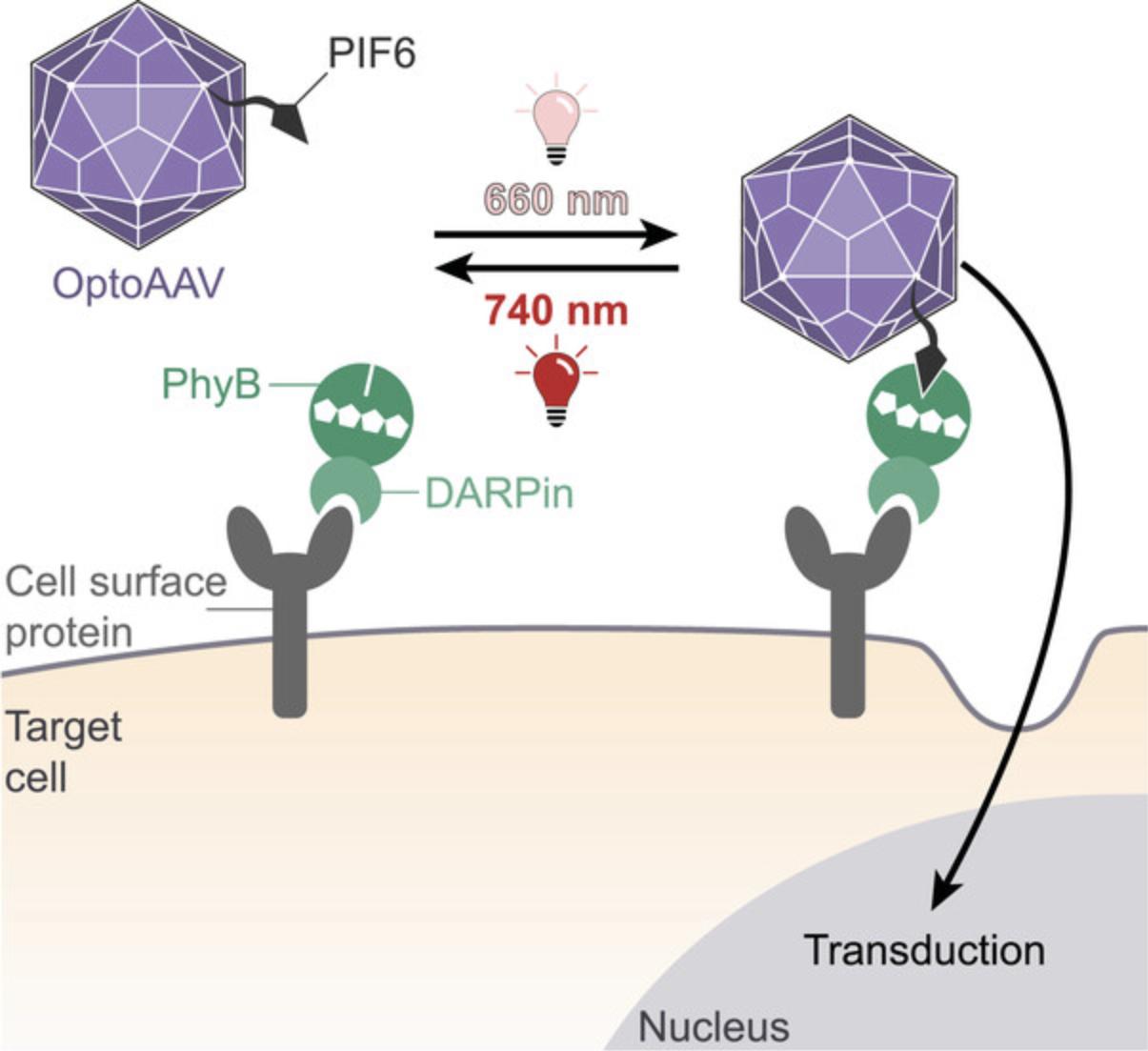
The OptoAAV technology provides multiple advantages compared to other light-controlled viral transduction methods (Hörner et al., 2021). First, as it controls viral transduction at the level of cell entry, it minimizes the impact on off-target cells. Second, it uses cell-compatible and tissue-penetrating red and far-red light of low intensity. Third, the sequential application of different OptoAAVs allows the spatially resolved transfer of different transgenes into different cells of the same culture. Fourth, the OptoAAV system can be easily controlled with LEDs and photomasks or with a conventional confocal microscope to allow single-cell resolution. Finally, it allows the transduction of both immortalized cell lines as well as primary cells (Hörner et al., 2021).
Critical Parameters
The performance of the OptoAAV system directly depends on the quality of both the OptoAAV and the adapter protein. Therefore, it is essential that the quality checks described in the analysis section of each protocol (Basic Protocols 1 and 2) are performed before applying the complete OptoAAV system (Basic Protocol 3 and Support Protocol). In case the OptoAAV system is not working despite positive quality checks, we suggest analysis of the functionality of both components in more detail. For the adapter protein, we suggest (1) measurement of the absorbance spectra after 660- and 740-nm illumination, (2) measurement of the light-dependent interaction with mVenus-PIF6 by size exclusion chromatography, (3) measurement of binding of the adapter protein to the target cells by flow cytometry utilizing the PhyB fluorescence (APC channel) or immunolabeling with a fluorescent anti-His6 antibody, and (4) analysis of the light-dependent recruitment of mVenus-PIF6 to the target cells in presence of the adapter protein by flow cytometry or confocal microscopy. For the OptoAAV, we suggest (1) analysis of the light-dependent binding to PhyB-coated beads and (2) analysis of background transduction of the target cells at high MOI. These experiments will allow the systematic identification of the reason, in case the OptoAAV system is not working as expected.
For the handling of the OptoAAV system, illumination is a critical parameter. The photoreceptor PhyB of the adapter protein is very light sensitive. Ambient room light will usually activate PhyB within seconds, in a similar fashion to 660-nm light. Consequently, even low-intensity room light results in substantial activation of the system. Therefore, always handle the OptoAAV system under dim green light as PhyB has its absorption minimum at ∼500 nm. However, high-intensity green light can also activate PhyB and thus the OptoAAV system. For efficient activation of the OptoAAV system, it is important to either use low-intensity red light (<10 µmol m-2 s-1) or to pulse high-intensity red light as PhyB is constantly shuttling between its active and inactive state under red light in a light intensity-dependent manner (Yousefi et al., 2019). This shuttling negatively impacts the binding of the OptoAAV to the cell surface. In the dark, PhyB is not constantly shuttling but activated PhyB reverts to its inactive state by thermal reversion with a half-life time of several hours. Consequently, pulsing of high-intensity red light is required to ensure maximal activation of the OptoAAV system. The wavelength of the inactivating far-red light should be selected to allow efficient inactivation of PhyB at low light intensity. Usually, LEDs with a peak wavelength of 740 nm are optimal for this purpose. However, some 740-nm LEDs have such a broad emission peak that the emitted lower wavelengths also result in PhyB activation. In this case, select LEDs with a narrow emission peak or with a higher peak wavelength (e.g., 760 nm). The transduction of the OptoAAV system under far-red light illumination should be similar to the transduction of the OptoAAV alone without adapter protein.
A further critical parameter for the OptoAAV system is the used MOI (multiplicity of infection, vector genomes per cell). In case the MOI is too low, no or only weak transduction will occur. In case the MOI is too high, background infection at 740-nm light will occur. Consequently, the optimal MOI is a tradeoff between high transduction under 660-nm light and low background transduction under 740-nm light and depends on the desired application. The optimal MOI depends further on the target cells and has to be optimized experimentally for each cell type.
Troubleshooting
For troubleshooting suggestions, see Table 1.
Problem | Possible cause | Solution |
---|---|---|
No band visible in Zn2+ staining | Low sensitivity | Increase exposure time. The bands are much weaker than usually imaged ethidium-bromide-stained DNA bands. |
No VP1 and PIF6-VP2 bands visible in Western blot | Low sensitivity; exposure time is adjusted to strong VP3 band | Increase exposure time. Use more sensitive ECL substrate (Femto substrate, Thermo Fisher Scientific, cat. no. 34095) |
Low OptoAAV titer | Contamination of plasmid DNA with RNA | Prepare new transfection-grade plasmid DNA |
OptoAAV system not working | Adapter protein not functional | Check functionality of adapter protein (see Critical Parameters). Repeat production. |
OptoAAV not functional | Check functionality of OptoAAV (see Critical Parameters). Repeat production. | |
Adapter protein is not compatible with target cells | Select adapter protein with strong binding to the surface of the target cells | |
Low transduction under 660-nm light | MOI too low | Increase MOI |
Low accessibility of PIF6 | Perform limited heat treatment as described in the protocol | |
Light intensity too high | Pulse light or reduce light intensity | |
High transduction under 740-nm light | MOI too high | Reduce MOI |
Activation by other light sources |
Reduce intensity of green light while handling the OptoAAV system. Increase intensity of 740-nm light. |
|
Far-red LED is also emitting activating light (e.g., broad emission peak) | Check LED emission spectrum/data sheet. Use LEDs with higher peak wavelength (e.g., 760 nm). | |
High background signal in microscopy images | Imaging of cellular autofluorescence. For viral vectors, transgene expression is usually much weaker than for transient transfection. |
Spectral imaging and linear unmixing to separate fluorescent signal from autofluorescence. Increase transgene (e.g., GFP) signal by immunofluorescence staining. |
Understanding Results
In Basic Protocol 1, the production, purification, and analysis of the adapter protein PhyB-DARPinEGFR is described. In Figure 1, representative results of the described SDS-PAGE analysis of purified PhyB-DARPinEGFR are shown. In the Zn2+ staining, the PhyB chromophore PCB is visualized whereas the Coomassie solution stains all proteins. The strong band slightly below the 100 kDa band of the protein ladder visible in both stainings is clearly identified as PhyB-DARPinEGFR (calculated molecular weight: 89.4 kDa). The Coomassie-staining reveals that purified PhyB-DARPinEGFR comes with some impurities and the single defined band in the Zn2+ staining shows the absence of PhyB-DARPinEGFR degradation products. The impurities are not expected to affect the performance of the OptoAAV system as transduction is usually performed in the presence of high amounts of BSA or FBS. For determination of the PhyB-DARPinEGFR concentration by Bradford assay, we neglected the impact of the impurities.
In Basic Protocol 2, the production, purification, and analysis of OptoAAV is described. Figure 3 shows representative results of the described Western blot analysis of iodixanol-purified OptoAAV using the B1 antibody specific for the viral capsid proteins VP1, VP2, and VP3. For comparison, unmodified wild-type (WT) AAV-2 was analyzed in parallel. The presence of the PIF6-VP2 band and the absence of the VP2 band in the OptoAAV lane demonstrates the successful modification of the viral capsid. The shift of the VP1 and VP3 bands towards higher molecular weight for OptoAAV compared to WT AAV-2 is caused by the mutations R585A and R588A in the capsid proteins (HSPG knockout; Münch et al., 2013).
In Basic Protocol 3, light-controlled transduction of A-431 cells by global illumination with 660- or 740-nm light is described and representative results of this experiment are depicted in Figure 4. Illumination with 660-nm light resulted in transduction of 64% of the cells compared to 1.7% under 740-nm light (37-fold increase). In the Support Protocol, the spatially resolved transduction of A-431 cells with two different OptoAAVs is described. Figure 5 shows a schematic overview of the experimental procedure and representative experimental results. Patterned illumination with 660-nm light using photomask 1 and photomask 2 resulted in spatially resolved transduction of the cells with OptoAAVGFP and OptoAAVmScarlet, respectively.
Time Considerations
Basic Protocol 1: 5 days. Day 1, Transformation: 2 hr. Day 2, Inoculation of expression culture and induction of protein production: 8 hr including 1 hr hands-on time. Day 3, Harvesting of expression culture: 1 hr. Day 4, Purification of PhyB-DARPinEGFR by IMAC: 10 hr. Day 5, Analysis of PhyB-DARPinEGFR: 4 hr.
Basic Protocol 2: 8 days. Day 1, Cell seeding: 1 hr. Day 2, Transfection with PEI: 8 hr including 2 hr hands-on time. Day 5, Harvesting of OptoAAV: 4 hr. Day 6, Purification of OptoAAV by iodixanol gradient ultracentrifugation: 6 hr. Day 7, Analysis of purified OptoAAV by Western blot and qPCR: 8 hr. Day 8, Analysis of purified OptoAAV by Western blot: 2 hr.
Basic Protocol 3: 4 days. Day 1, Cell seeding: 1 hr. Day 2, Light-controlled viral transduction: 4 hr. Day 4, Analysis of light-controlled viral transduction by flow cytometry: 2 hr.
Support Protocol: 4 days. Day 1, Cell seeding: 1 hr. Day 2, Spatially-resolved viral transduction: 6 hr. Day 3, Reduction of temperature: 5 min. Day 4, Fixation of cells and analysis by fluorescence microscopy: 6 hr.
Acknowledgments
The authors are grateful to A. Hartley, E. Wiedtke, and D. Grimm (Heidelberg University, Germany) for providing plasmids pCMVgfp and pCMVmScarlet and for helpful advice. We would like to thank H. Büning (Hannover Medical School, Germany) for providing plasmid pRCVP2koA and for helpful advice. This work was supported by the Deutsche Forschungsgemeinschaft (DFG, German Research Foundation) under Germany's Excellence Strategy CIBSS - EXC-2189 - Project ID: 390939984 and under the Excellence Initiative of the German Federal and State Governments - EXC- 294, and in part by the Ministry for Science, Research and Arts of the State of Baden-Württemberg.
Open access funding enabled and organized by Projekt DEAL.
Author Contributions
Maximilian Hörner : Conceptualization, investigation, methodology, project administration, visualization, writing original draft; Wilfried Weber : Conceptualization, funding acquisition, supervision, writing, review, and editing.
Conflict of Interest
The University of Freiburg has filed a patent application covering the technology described here of which M.H. and W.W. are inventors.
Open Research
Data Availability Statement
Data sharing is not applicable to this article as no new data were created or analyzed in this study.
Literature Cited
- Bonsted, A., Engesaeter, B. O., Hogset, A., Maelandsmo, G. M., Prasmickaite, L., D'Oliveira, C., … Berg, K. (2006). Photochemically enhanced transduction of polymer-complexed adenovirus targeted to the epidermal growth factor receptor. Journal of Gene Medicine , 8(3, 286–297. doi: 10.1002/jgm.853.
- Bonsted, A., Hogset, A., Hoover, F., & Berg, K. (2005). Photochemical enhancement of gene delivery to glioblastoma cells is dependent on the vector applied. Anticancer Research , 25(1A), 291–297.
- Bugaj, L. J., & Lim, W. A. (2019). High-throughput multicolor optogenetics in microwell plates. Nature Protocols , 14(7), 2205–2228. doi: 10.1038/s41596-019-0178-y.
- Goater, J., Müller, R., Kollias, G., Firestein, G. S., Sanz, I., O'Keefe, R. J., & Schwarz, E. M. (2000). Empirical advantages of adeno associated viral vectors for in vivo gene therapy for arthritis. Journal of Rheumatology , 27(4), 983–989.
- Gomez, E. J., Gerhardt, K., Judd, J., Tabor, J. J., & Suh, J. (2016). Light-activated nuclear translocation of adeno-associated virus nanoparticles using phytochrome B for enhanced, tunable, and spatially programmable gene delivery. ACS Nano , 10(1), 225–237. doi: 10.1021/acsnano.5b05558.
- Hagihara, Y., Sakamoto, A., Tokuda, T., Yamashita, T., Ikemoto, S., Kimura, A., … Mizuguchi, H. (2020). Photoactivatable oncolytic adenovirus for optogenetic cancer therapy. Cell Death & Disease, 11(7), 570. doi: 10.1038/s41419-020-02782-6.
- Hogset, A., Engesaeter, B. O., Prasmickaite, L., Berg, K., Fodstad, O., & Maelandsmo, G. M. (2002). Light-induced adenovirus gene transfer, an efficient and specific gene delivery technology for cancer gene therapy. Cancer Gene Therapy , 9(4), 365–371. doi: 10.1038/sj.cgt.7700447.
- Hörner, M., Gerhardt, K., Salavei, P., Hoess, P., Harrer, D., Kaiser, J., … Weber, W. (2019). Production of phytochromes by high-cell-density E. coli fermentation. ACS Synthetic Biology , 8(10), 2442–2450. doi: 10.1021/acssynbio.9b00267.
- Hörner, M., Jerez-Longres, C., Hudek, A., Hook, S., Yousefi, O. S., Schamel, W. W. A., … Weber, W. (2021). Spatiotemporally confined red light-controlled gene delivery at single-cell resolution using adeno-associated viral vectors. Science Advances , 7(25). doi: 10.1126/sciadv.abf0797.
- Ito, H., Goater, J. J., Tiyapatanaputi, P., Rubery, P. T., O'Keefe, R. J., & Schwarz, E. M. (2004). Light-activated gene transduction of recombinant adeno-associated virus in human mesenchymal stem cells. Gene Therapy , 11(1), 34–41. doi: 10.1038/sj.gt.3302102.
- Müller, K., Zurbriggen, M. D., & Weber, W. (2014). Control of gene expression using a red- and far-red light-responsive bi-stable toggle switch. Nature Protocols , 9(3), 622–632. doi: 10.1038/nprot.2014.038.
- Münch, R. C., Janicki, H., Volker, I., Rasbach, A., Hallek, M., Büning, H., & Buchholz, C. J. (2013). Displaying high-affinity ligands on adeno-associated viral vectors enables tumor cell-specific and safe gene transfer. Molecular Therapy , 21(1), 109–118. doi: 10.1038/mt.2012.186.
- Pandori, M. W., Hobson, D. A., Olejnik, J., Krzymanska-Olejnik, E., Rothschild, K. J., Palmer, A. A., … Sano, T. (2002). Photochemical control of the infectivity of adenoviral vectors using a novel photocleavable biotinylation reagent. Chemistry & Biology, 9(5), 567–573. doi: 10.1016/s1074-5521(02)00135-7.
- Pandori, M. W., & Sano, T. (2000). Photoactivatable retroviral vectors: A strategy for targeted gene delivery. Gene Therapy , 7(23), 1999–2006. doi: 10.1038/sj.gt.3301338.
- Tahara, M., Takishima, Y., Miyamoto, S., Nakatsu, Y., Someya, K., Sato, M., … Takeda, M. (2019). Photocontrollable mononegaviruses. Proceedings of the National Academy of Sciences of the United States of America , 116(24), 11587–11589. doi: 10.1073/pnas.1906531116.
- Thomas, O. S., Hörner, M., & Weber, W. (2020). A graphical user interface to design high-throughput optogenetic experiments with the optoPlate-96. Nature Protocols , 15(9), 2785–2787. doi: 10.1038/s41596-020-0349-x.
- Ulrich-Vinther, M., Maloney, M. D., Goater, J. J., Soballe, K., Goldring, M. B., O'Keefe, R. J., & Schwarz, E. M. (2002). Light-activated gene transduction enhances adeno-associated virus vector-mediated gene expression in human articular chondrocytes. Arthritis & Rheumatism, 46(8), 2095–2104. doi: 10.1002/art.10433.
- Wang, Y., Bruggeman, K. F., Franks, S., Gautam, V., Hodgetts, S. I., Harvey, A. R., … Nisbet, D. R. (2021). Is viral vector gene delivery more effective using biomaterials? Advanced Healthcare Materials , 10(1), e2001238. doi: 10.1002/adhm.202001238.
- Wang, Y., Li, S., Tian, Z., Sun, J., Liang, S., Zhang, B., … Zhou, D. (2019). Generation of a caged lentiviral vector through an unnatural amino acid for photo-switchable transduction. Nucleic Acids Research , 47(19), e114. doi: 10.1093/nar/gkz659.
- Xiao, X., Li, J., & Samulski, R. J. (1998). Production of high-titer recombinant adeno-associated virus vectors in the absence of helper adenovirus. Journal of Virology , 72(3), 2224–2232. doi: 10.1128/JVI.72.3.2224-2232.1998.
- Yousefi, O. S., Gunther, M., Hörner, M., Chalupsky, J., Wess, M., Brandl, S. M., … Schamel, W. W. (2019). Optogenetic control shows that kinetic proofreading regulates the activity of the T cell receptor. eLife , 8, e42475. doi: 10.7554/eLife.42475.
- Zolotukhin, S., Byrne, B. J., Mason, E., Zolotukhin, I., Potter, M., Chesnut, K., … Muzyczka, N. (1999). Recombinant adeno-associated virus purification using novel methods improves infectious titer and yield. Gene Therapy , 6(6), 973–985. doi: 10.1038/sj.gt.3300938.
Key References
- Hörner et al. (2021). See above
This study describes the OptoAAV technology for which the detailed experimental protocol is provided in this article.