Minimizing Variability in Developmental Fear Studies in Mice: Toward Improved Replicability in the Field
Hanista Premachandran, Hanista Premachandran, Jennifer Wilkin, Jennifer Wilkin, Maithe Arruda-Carvalho, Maithe Arruda-Carvalho
Abstract
In rodents, the first weeks of postnatal life feature remarkable changes in fear memory acquisition, retention, extinction, and discrimination. Early development is also marked by profound changes in brain circuits underlying fear memory processing, with heightened sensitivity to environmental influences and stress, providing a powerful model to study the intersection between brain structure, function, and the impacts of stress. Nevertheless, difficulties related to breeding and housing young rodents, preweaning manipulations, and potential increased variability within that population pose considerable challenges to developmental fear research. Here we discuss several factors that may promote variability in studies examining fear conditioning in young rodents and provide recommendations to increase replicability. We focus primarily on experimental conditions, design, and analysis of rodent fear data, with an emphasis on mouse studies. The convergence of anatomical, synaptic, physiological, and behavioral changes during early life may increase variability, but careful practice and transparency in reporting may improve rigor and consensus in the field. © 2024 The Authors. Current Protocols published by Wiley Periodicals LLC.
INTRODUCTION
Fear memory processing is crucial for threat response and survival. In rodents, fear memories are often studied using Pavlovian fear conditioning, whereby a conditioned stimulus (CS, e.g., a neutral tone) is paired with an unconditioned stimulus (US, e.g., foot shock) to generate a CS-US association. The CS-US association is later assessed by presentation of the CS alone, which triggers the innate fear response of freezing, defined as the absence of all movement except for breathing (Blanchard & Blanchard, 1969; Fanselow, 1980). Given the importance of fear learning to survival, it is not surprising that rodents can form fear associations from a very early age. Despite its early onset, multiple aspects of fear processing continue to change across early postnatal development in mice and rats, including the ability to form, refine, inhibit, and retain fear memories (Akers et al., 2012, 2014; Alberini & Travaglia, 2017; Baker & Richardson, 2015; Bath et al., 2016; Campbell & Ampuero, 1985; Campbell & Campbell, 1962; Campbell & Spear, 1972; Ganella & Kim, 2014; Gogolla et al., 2009; Guskjolen et al., 2018; Josselyn & Frankland, 2012; Kim et al., 2006, 2009; Kim & Richardson, 2007a,b, 2008; Kucharski & Spear, 1984; Moriceau & Sullivan, 2006; Park et al., 2017b; Park, Ganella, Perry et al., 2020; Pattwell et al., 2012, 2016; Revillo et al., 2015; Rudy & Morledge, 1994; Samifanni et al., 2021; Sullivan et al., 2000; Tallot et al., 2016). Importantly, these changes occur against a backdrop of other developmental milestones, including (1) leaving the nest, increasing both exploration and exposure to threats, (2) anatomical and synaptic changes in the brain, and (3) heightened sensitivity to environmental influences and stress (Bell, 2018; Brenhouse & Andersen, 2011; Brust et al., 2015; Knudsen, 2004; Livia Terranova & Laviola, 1995; Sachser et al., 2020). The confluence of these factors imposes serious challenges and potentially confounds the study of fear processing during early life. Here we provide a brief overview of developmental differences in fear processing in rodents, followed by a discussion of some potential underlying factors, providing recommendations aimed at minimizing experimental variability, with a focus on mouse studies (also see the exemplary overview by Cowan & Richardson, 2018).
NOTE : All protocols involving live animals must be reviewed and approved by an Institutional Animal Care and Use Committee (IACUC) and must conform to government regulations for the care and use of laboratory animals.
DEVELOPMENTAL DIFFERENCES IN FEAR PROCESSING IN RODENTS
Infancy and Juvenility
Decades of prior research suggest that fear processing is developmentally regulated (Akers et al., 2012, 2014; Alberini & Travaglia, 2017; Campbell & Campbell, 1962; Campbell & Spear, 1972; Ganella & Kim, 2014; Guskjolen et al., 2018; Herry et al., 2010; Josselyn & Frankland, 2012; Kim et al., 2006, 2009; Kim & Richardson, 2008; Li et al., 2012; Li et al., 2018; Moriceau & Sullivan, 2006; Park et al., 2017b; Park, Ganella, Perry et al., 2020; Pattwell et al., 2012; Revillo et al., 2015; Rudy & Morledge, 1994; Samifanni et al., 2021; Sullivan et al., 2000; Tallot et al., 2016). Freezing behavior is first expressed during infancy in rats (by postnatal day [P] 10; Takahashi, 1994), a time in which they begin to venture outside of the nest and show increased exploration (Bolles & Woods, 1964). Seminal work in infant rats has shown that rats up to P10 will approach an odor that was previously paired with an aversive foot shock, but from P10 onwards they begin to avoid the odor and demonstrate aversion learning (Moriceau & Sullivan, 2004, 2006; Sullivan et al., 2000; Sullivan & Wilson, 1994; Tallot et al., 2016), exemplifying the nonlinear changes that shape the emergence of fear learning in rodents.
During juvenility (often noted as ∼P18-29; Bell, 2018; Cowan & Richardson, 2018), mice and rats venture out of the nest more frequently, thereby increasing encounters with threatening stimuli (Bell, 2018; Brust et al., 2015). As a result, this is a crucial time for fear-related systems to come online and ensure survival. Accordingly, juvenility marks the onset of the acquisition and retention of life-long or persistent fear memories (Akers et al., 2012, 2014; Alberini & Travaglia, 2017; Campbell & Ampuero, 1985; Campbell & Campbell, 1962; Guskjolen et al., 2018; Josselyn & Frankland, 2012; Nieves et al., 2020; Revillo et al., 2015; Samifanni et al., 2021). Juvenility also features a transition in the way rodents extinguish fear memories (Callaghan & Richardson, 2013; Gogolla et al., 2009; Kim & Richardson, 2007a,b; Park et al., 2017b; Park, Ganella, Perry et al., 2020; Yap & Richardson, 2007). Specifically, when infant rodents undergo fear extinction there is a permanent suppression of the fear response (immature-type extinction; Gogolla et al., 2009; Kim & Richardson, 2007a,b; Li et al., 2018; Park et al., 2017b; Park, Ganella, Perry et al., 2020; Yap & Richardson, 2007); however, starting from juvenility, extinction ceases to be so permanent and animals start to display a return of the fear memory over time, even spontaneously (adult-type extinction; Bouton & Bolles, 1979a,b; Corcoran & Maren, 2004; Herry et al., 2010; Myers & Davis, 2007; Pape & Pare, 2010; Quirk & Mueller, 2008; Rescorla & Heth, 1975). This transition between immature and adult extinction profiles occurs sometime between P16 and P23 in mice (Gogolla et al., 2009) and between P17 and P18 in rats (Kim & Richardson, 2007a,b; Park et al., 2017b; Park, Ganella, Perry et al., 2020; Revillo et al., 2016; Revillo, Paglini, & Arias, 2014). Juvenility is also marked by an increase in the precision of contextual fear memories, which reach adult levels of contextual discrimination at P24 in mice (Ramsaran et al., 2018, 2023).
Puberty, Adolescence, and Sex Differences
Even though juvenility encompasses key transitions in fear processing systems that coincide with ethological changes in threat exposure, adolescence (P30-55; Cowan & Richardson, 2018) has been the prime focus of rodent developmental fear studies. The transition between juvenility and adolescence is accompanied by several physiological and behavioral changes, including endocrine changes marking the onset of puberty and sexual maturity as well as increased risk-taking behaviors (Schneider, 2013; Spear, 2000; Vetter-O'Hagen & Spear, 2012; Walker et al., 2017). Although the first surge of gonadal hormones occurs during the perinatal period (1 week before and after birth), a second surge occurs during puberty (McCarthy et al., 2017), which often overlaps with adolescence (Vetter-O'Hagen & Spear, 2012). Importantly, as circulating hormones in early development alter and shape neural networks, the onset of puberty may bring further sex-specific changes in anatomy, circuitry, and behavior. This is particularly relevant to fear processing because pubertal hormones can influence the formation, extinction, and generalization of fear memories (Colón et al., 2023; Crestani et al., 2022; McDermott et al., 2012; Perry et al., 2020). Notably, the onset of puberty is known to vary by sex, which may increase variability in developmental fear research. In general, female rodents have an earlier onset of puberty compared to males, similar to humans (Schneider, 2013). Female mice show external signs of puberty around P26-29 and female rats by P30-39 (Piekarski et al., 2017; Schneider, 2013; Vetter-O'Hagen & Spear, 2012). In contrast, male mice first show pubertal markers around P30 and male rats around P40-45 (Koss et al., 2015; Schneider, 2013; Vetter-O'Hagen & Spear, 2012). Given that females have an earlier puberty onset, it is likely that gonadal hormones may contribute to transient and/or long-lasting sex differences in systems encompassing fear processing around adolescence. Indeed, several lines of evidence suggest that specific neural correlates of fear processing mature earlier in female rodents (for review, see Premachandran et al., 2020). As one example, female rats display an earlier transition to the adult-type extinction system compared to males (Park et al., 2017b), but this timeline has not been assessed in female mice.
Sex differences are also found in fear extinction in early life. While many studies report a deficit in extinction learning and/or retrieval in adolescent males (Baker & Richardson, 2015; Baker-Andresen et al., 2013; Callaghan & Richardson, 2012b; Ganella et al., 2017; McCallum et al., 2010; Pattwell et al., 2012, 2016; but also see Experimental Design section), adolescent female rats show improved extinction compared to their adult counterparts (McCormick et al., 2013), suggesting a potential sex difference in extinction learning during adolescence. Importantly, Perry et al. (2020) reported that adolescent female rats in proestrus or metestrus/diestrus display impaired extinction compared to adolescent males (and females in estrus), pointing to a role for the estrous cycle in regulating extinction during adolescence. Together, these studies emphasize a need to expand research on sex differences in adolescent fear extinction and highlight the importance of monitoring estrous cycle in developmental fear experiments.
Given the considerable influence of gonadal hormones in fear behavior during adolescence and adulthood (Chang et al., 2009; Colón et al., 2023; Crestani et al., 2022; Gupta et al., 2001; Jasnow et al., 2006; Lebron-Milad & Milad, 2012; McDermott et al., 2012; Milad et al., 2009; Perry et al., 2020), it is expected that the onset of puberty between late juvenility and early adolescence will contribute to variability in fear processing. Importantly, this timing can also be influenced by strain in both mice and rats (Bell, 2018; Keeley et al., 2015; Nelson et al., 1990). Strain and substrain differences in fear behavior have been reported in both adolescent and adult rodents (Balogh & Wehner, 2003; Camp et al., 2012; Cazares et al., 2019; Eltokhi et al., 2020; Hefner et al., 2008; MacPherson et al., 2013; Owen et al., 1997). For example, C57 substrains C57BL/6J (Jackson Laboratories), C57BL/6NCrl (Charles River Laboratories), and C57BL/6NHsd (Harlan Sprague-Dawley) show notable differences in fear behavior in adulthood, especially in contextual freezing levels (Bryant et al., 2008; Radulovic et al., 1998; Siegmund et al., 2005; Siegmund & Wotjak, 2007; Stiedl et al., 1999). Due to the interplay between strain differences and pubertal onset, strain differences in fear processing may be potentiated during adolescence.
Maturation of Neural Correlates of Fear
The aforementioned developmental changes in fear behavior coincide with the maturation of the brain circuits that support them. The first four postnatal weeks are marked by dramatic anatomical and synaptic changes in rodent brain regions that mediate fear processing, such as the amygdala, hippocampus (HPC), and medial prefrontal cortex (mPFC) (Arruda-Carvalho et al., 2017; Baker et al., 2017; Bessières et al., 2019; Blair et al., 2013; Bosch & Ehrlich, 2015; Caballero et al., 2016; Caballero & Tseng, 2016; Donato et al., 2021; Gerhard et al., 2021; Hartley & Lee, 2015; Jia et al., 2018; Kalemaki et al., 2022; King et al., 2014; Klune et al., 2021; Kolb et al., 2012; Koss et al., 2014; Kroon et al., 2019; Premachandran et al., 2020; Ramsaran et al., 2023; Rubinow & Juraska, 2009; Swann et al., 1989; Travaglia, Bisaz, Cruz et al., 2016; Travaglia, Bisaz, Sweet et al., 2016; Zimmermann et al., 2019). Interestingly, these brain regions support different aspects of fear processing and also mature at different rates. The amygdala is crucial for learning the association between CS and US (Cousens & Otto, 1998; Ehrlich et al., 2009; Maren, 2003; Maren et al., 1996; Muller et al., 1997; Orsini & Maren, 2012; Phelps & LeDoux, 2005) and is one of the first to mature, showing activity during fear behaviors from infancy in rats (Moriceau & Sullivan, 2004, 2006; Sullivan et al., 2000; Tallot et al., 2016). The HPC is essential for contextual fear associations (Debiec et al., 2002; Fanselow, 2000; Matus-Amat et al., 2004; Phelps & LeDoux, 2005; Phillips & LeDoux, 1992; Rudy et al., 2002; Sanders et al., 2003; Xu et al., 2016) and is recruited during juvenility to support contextual fear learning (Campbell & Campbell, 1962; Raineki et al., 2010; Rudy & Morledge, 1994; Schiffino et al., 2011). The mPFC plays an important role in fear expression and extinction (Arruda-Carvalho & Clem, 2015; Burgos-Robles et al., 2009; Corcoran & Quirk, 2007; Herry et al., 2008, 2010; Ledoux, 2000; Milad & Quirk, 2002; Miller & Cohen, 2001; Mukherjee & Caroni, 2018; Rozeske et al., 2015; Sierra-Mercado et al., 2006; Vidal-Gonzalez et al., 2006) and is relatively late to mature (Casey et al., 2013; Klune et al., 2021; van Eden & Uylings, 1985; Zimmermann et al., 2023), reaching adult levels of activity around adolescence (Arruda-Carvalho et al., 2017; Kalemaki et al., 2022), which coincides with the onset of its involvement in auditory fear retrieval (Li et al., 2012).
Remarkably, these brain regions are also highly sensitive to stress (Akirav & Maroun, 2007; Arnsten, 2009; Cohen et al., 2013; Ferrara et al., 2021; Honeycutt et al., 2020; Johnson et al., 2018; Liu et al., 2017; McEwen et al., 2016; McEwen & Morrison, 2013; Roozendaal et al., 2009). Stress experienced in early life exerts greater impact than that experienced in adulthood (Cotella et al., 2019; Jankord et al., 2011; Romeo, 2010; Romeo & McEwen, 2006; Yohn & Blendy, 2017), including impacts on emotional learning trajectories. For instance, injection of corticosterone leads to early recruitment of the amygdala in the acquisition of odor aversion learning (Moriceau & Sullivan, 2004, 2006). Additionally, early life stress accelerates HPC maturation and the emergence of fear behaviors dependent on the HPC (e.g., earlier onset of contextual fear suppression; Bath et al., 2016), mPFC, and amygdala (e.g., earlier onset of adult-type extinction; Callaghan et al., 2014; Callaghan & Richardson, 2011, 2012b, 2013, 2014; Callaghan & Tottenham, 2016; Cowan et al., 2013).
Overall, these studies show that early development is marked by a convergence of anatomical, synaptic, physiological, and behavioral changes, as well as heightened stress sensitivity within fear circuits and associated behavior. While these changes may add variability and impose unique challenges to the study of emotional learning during early life, they also provide a unique and valuable prism for the study of brain-behavior dynamics and the consequences of external influences. Below we highlight a few of the specific factors contributing to variability in rodent developmental fear studies, focusing on breeding and housing conditions, experimental design, and analysis of fear behavioral data in mice. While others have made important contributions to this topic (Bisby et al., 2021; Cowan & Richardson, 2018) and our list is by no means exhaustive, we provide some recommendations aimed at fostering consensus, reducing variability, and increasing replicability when running Pavlovian fear conditioning experiments with young mice.
BREEDING AND HOUSING CONDITIONS
The conditions under which rodents enter and are kept in an animal facility—including how they are bred, housed (bedding, nesting, number of cagemates, among others), and manipulated—have a profound impact on many experimental outcomes, and fear behavior is no exception (Fig. 1). Furthermore, given that early life spans some of the most significant changes in housing conditions (e.g., birth, separation from the dam at weaning), it is conceivable that the impact of housing conditions will be greater in studies sampling early postnatal ages compared to those conducted in adulthood. Below we discuss a few potential sources of variability related to the way animals arrive at the facility, how they are housed, and how/when they are separated from the dam (weaning).
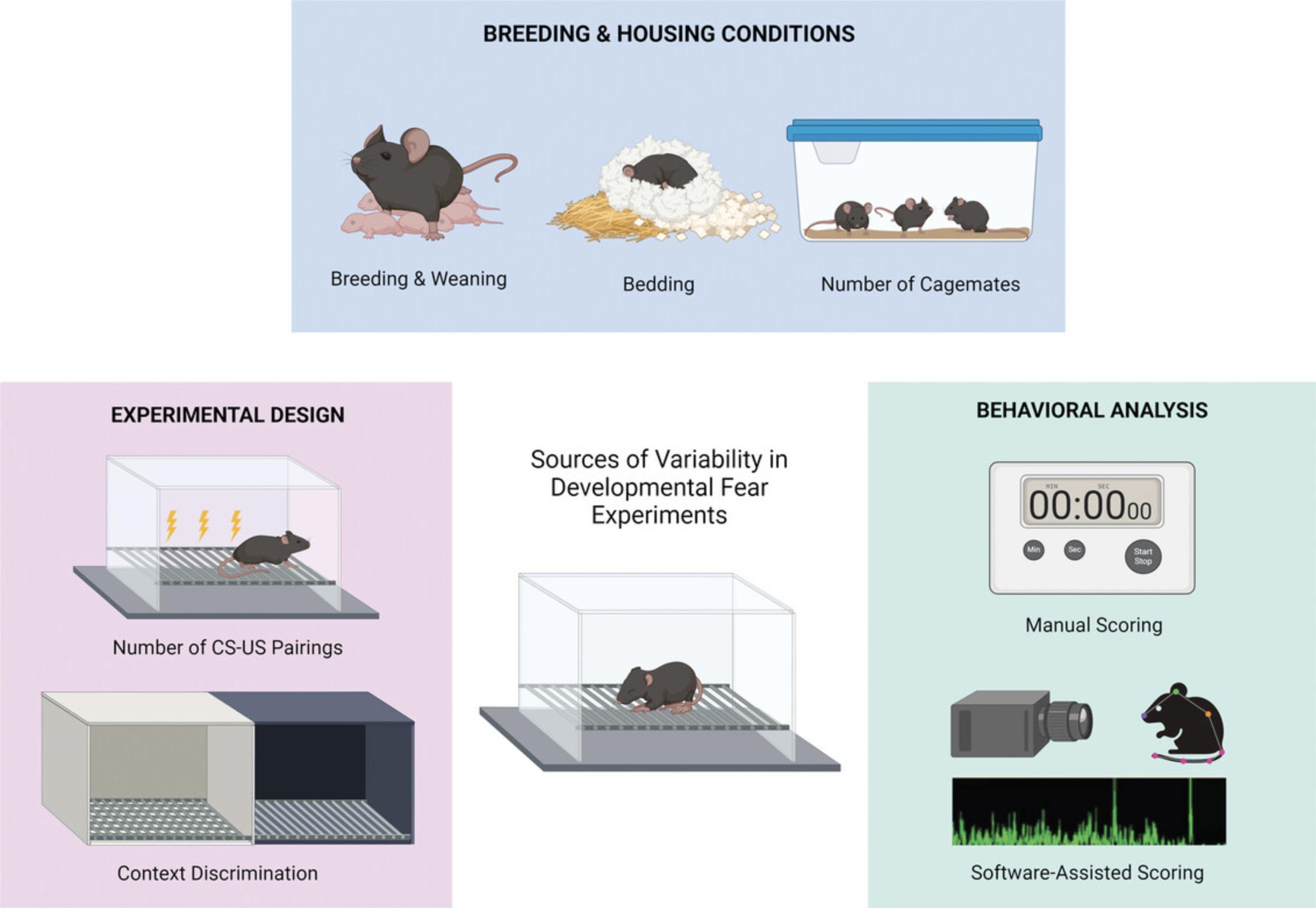
In-house Breeding Versus Animal Shipping
As many developmental fear experiments span ages close to weaning, they predominantly use in-house breeding, i.e., the animals are bred in the same animal facility in which experiments are conducted (Hampshire & Davis, 2005). In-house breeding minimizes transportation stress and facilitates access to early developmental stages. Despite these advantages, the time needed for each breeding cycle and other intrinsic challenges of colony management may reduce the rate at which experimental animals become available, delaying research progress. When working with juvenile or adolescent rodents, an alternative is to purchase animals directly from vendors and allow them to acclimate to the facility before testing (e.g., Ferrara et al., 2020; Hefner & Holmes, 2007; Selleck et al., 2018). It is important to note that, since this strategy requires that pups be weaned at the vendor facility prior to shipping, this approach may add variability in terms of handling and weaning. Additionally, when the animal arrival date coincides with weaning age, the stress of weaning may be conflated with that of shipping. Given the stress of shipping (Laroche et al., 2009), and that early life stress can increase fear and anxiety responses in adulthood (Malter Cohen et al., 2013; Moriceau et al., 2009; Oomen et al., 2010), the timing of shipping (e.g., juvenility versus adulthood) may be a consideration. Allowing animals to acclimate for a few days prior to behavior assessment is highly recommended to mitigate the acute effects of shipping stress. Critically, control animals must undergo the same shipping procedures as the experimental group to minimize the effects of breeding method on between-group comparisons. Nevertheless, these differences in early life experiences should be taken into account when comparing studies that use different breeding methods.
Bedding
Regardless of source, all rodents in the animal facility are placed in a cage with bedding. Although often overlooked as a variable, bedding type varies significantly between animal facilities and can have an effect on fear behavior. For example, in female (but not male) adult mice, bedding type influences freezing during contextual fear training and extinction, with small wood bedding resulting in lower freezing compared to large paper bedding (Matsuda, 2023). This may be especially relevant during development, as the smaller paws of young mice could lead them to experience bedding coarseness differently than adults. Moreover, bedding type affects the mechanical withdrawal reflex in mice, with coarse bedding reducing sensitivity (Moehring et al., 2016), which could affect pain sensitivity during foot shock. Bedding type also affects the development of mechanical hyperalgesia and chemical hypersensitivity in rats, with coarse bedding reducing pain sensitivity compared to fine sawdust (Robinson et al., 2004). Therefore, differences in bedding type could be a major contributor to low replicability of fear studies across animal facilities, even with the same species/strain and experimental design. Furthermore, some labs use aspen (Park, Ganella, Perry et al., 2020), wood chip (Ganella et al., 2016, 2017; Park et al., 2017b; Perry et al., 2020) or corncob (Bisby, Richardson et al., 2020) bedding as part of the context in the fear chamber itself (Kim et al., 2011; Kim & Richardson, 2007a,b; Yap & Richardson, 2007). Although not standard practice, including information on bedding type and size might help inform inconsistencies and increase replicability in the field.
Weaning
Weaning, a time when the litter is separated from the mother and placed in a new cage, is a significant period in an animal's life and plays an important role in physiology, behavior, and neurological development (Berry et al., 2012; Kanari et al., 2005; Ladd et al., 1996; Terranova & Laviola, 2001). The standard age of weaning in most rodent facilities is P21 (Bechard & Mason, 2010), and is therefore an important variable to consider when conducting developmental studies that sample adjacent ages. It is important to remark that weaning is an artificial procedure, since maternal care in the wild continues well beyond P21, with nursing in both rats and mice extending to P27-28 (König & Markl, 1987; Mendl & Paul, 1990; Oštádalová & Babický, 2012) and young mice dispersing from the mother around P40 (Berdoy & Drickamer, 2007). Even in a laboratory setting, if juvenile mice are provided with an alternative cage but still have access to the dam, pups continue to visit and receive maternal care up until P35 (Bechard & Mason, 2010). This is in stark contrast to the abrupt and permanent nature of weaning in an animal facility.
Several studies show that the timing of weaning, either before (early weaning) or after (late weaning) P21, affects a number of behaviors and physiological processes. Early weaning (typically between P14 and P16) is considered a form of early-life stress and robustly affects aggression, feeding behavior, anxiety, and social interaction (dos Santos Oliveira et al., 2011; Ishikawa et al., 2015; Ito et al., 2006; Kikusui et al., 2004, 2006, 2009; Kodama et al., 2008; Nakamura et al., 2003; Ono et al., 2008; Shimozuru et al., 2007; Takita & Kikusui, 2016). Early weaning can also lead to increased stereotypy, believed to represent an effort to return to the mother (Latham & Mason, 2008; Würbel & Stauffacher, 1997). Notably, some studies suggest that the effects of early weaning may be sex-specific, with early weaning males (but not females) showing increased glucocorticoid receptor expression and higher basal corticosterone levels even in adulthood (Kikusui et al., 2006). Further, female mice that are weaned early proceed to wean their own pups earlier (Curley et al., 2009), emphasizing the long-term effects of this manipulation. Interestingly, some of the endocrine and behavioral effects of early weaning have also been reported in animals with low body weight, with higher weaning weight being positively associated with adult reproductive success, especially in males (Krackow, 1993; Krackow & Hoeck, 1989). These data emphasize weaning weight as a potentially influential metric that should be recorded and correlated with future behavioral performance.
When it comes to fear processing, early weaning increases freezing during auditory fear retrieval (Kreiker et al., 2021), extinction training, and extinction retrieval (Mogi et al., 2016). Interestingly, early weaning also led to lower paired-pulse facilitation in the mPFC-amygdala pathway (Takita & Kikusui, 2016), which is strongly implicated in fear memory processing (Arruda-Carvalho & Clem, 2014; Bukalo et al., 2015; Cho et al., 2013; Do-Monte et al., 2015; Duvarci & Pare, 2014; Gunduz-Cinar et al., 2023; Herry et al., 2008; Hübner et al., 2014; Marek et al., 2013; Quirk & Mueller, 2008; Sotres-Bayon & Quirk, 2010; Stafford et al., 2013). In contrast, late weaning (usually P25 or later) leads to higher levels of social behavior (Curley et al., 2009), increased exploration, and reduced anxiety-like behavior (Richter et al., 2016). Nevertheless, the effects of late weaning on fear processing remain under-investigated. Despite an absence of these data, the broad effects of either early or late weaning described above make it clear that weaning is an important variable in the experimental design of developmental fear studies, particularly when sampling ages close to P21. If using a range of weaning ages (e.g., P21 ± 1), keeping the interval between weaning and behavior constant will likely help decrease variability in behavioral outcomes. As a general rule, limiting the age range within a sampled experimental group to ±1 or 2 days would further reduce variability associated with individual and sex differences in development, including those related to the onset of puberty.
One way to avoid the potential impacts of weaning is to postpone it until after the experimental timeline is complete. Juvenile (P25) rats that were not weaned show higher overall contextual freezing than those weaned at P21 (Park et al., 2017a). Interestingly, age-dependent differences in fear extinction are observed even in the absence of weaning (Gogolla et al., 2009; Kim & Richardson, 2007b; Yap & Richardson, 2007), but whether or not weaning alters their timing is unclear. While these studies point to eliminating weaning as an appealing strategy to minimize stress and variability, its application is restricted in age range, as most animal facilities will not allow late weaning past adolescence.
Considering the findings above, there is significant evidence that weaning affects fear conditioning and retrieval, and may differentially impact males and females. Differences in weaning procedures (and its proximity to the ages tested) across labs likely adds variability to developmental fear studies. Research directly comparing the effects of the timing of weaning on fear behavior conducted within the same facility and with identical experimental conditions would increase insight into the degree of its influence on fear memory outputs. At a minimum, recognition of weaning as a major variable and transparency in reporting of weaning procedures would improve replicability.
Animals Per Cage
The stress of weaning is predominantly attributed to separation from the dam, but it is also accompanied by another major change: reduction of the total number of animals that occupy the cage. Infant and juvenile rodents are initially kept with the dam in large groups of up to 14 pups, at an average of 6-8 in a lab setting (Jalali et al., 2016). Some studies cull litters to a certain size shortly after parturition—e.g., 8-10 pups (Brasser & Spear, 2004; Kreiker et al., 2021), 9 pups (Carew & Rudy, 1991), or 10 pups (Revillo, Castello, et al., 2014)—in order to better control for litter effects (for a recommendation to standardize litter sizes as early as possible, see Cowan & Richardson, 2018). Following weaning, the standard procedure in animal facilities is to house same-sex littermates together in groups of 3-5 (with considerable variation, see below).
Several studies point to the number of animals per cage as having a significant impact on behavior, including fear. Solo-housing for extended periods of time can lead to enhanced contextual fear responses and impaired fear extinction (Pibiri et al., 2008). On the other hand, overcrowding is considered a form of chronic social stress that increases anxiety-like behaviors in mice (Lin et al., 2015; Reiss et al., 2007). Interestingly, stress (including overcrowding) biases the sex-ratio of the progeny of stressed dams toward more females (Trivers & Willard, 1973), a parameter that should be monitored when conducting in-house breeding.
In Canada, animal care guidelines (CCAC, 2022) state a standard group size for mice as 4-5 per cage, with cages harboring at least 100 cm2 (15.5 in2) floor space per mouse, with studies emphasizing the importance of sufficient space requirements for mouse welfare (Bailoo et al., 2018; Makowska et al., 2019). In mouse developmental fear studies, cage allotment tends to cluster around 2-5 mice (Gerhard & Meyer, 2021; Lanjewar et al., 2023; Samifanni et al., 2021), though some limit the maximum to 4 animals per cage (Hefner & Holmes, 2007). Rat developmental studies feature greater variability in the number of animals per cage, with some reaching significantly higher numbers than most mouse studies. Ranges include a maximum of 3 (Ferrara et al., 2020) or 4 (Chocyk et al., 2014) animals per cage to groups of 5-6 (Perry et al., 2020) or 6 (Ganella et al., 2017). Some studies use up to 4-8 (McCallum et al., 2010; Yap & Richardson, 2007; Zimmermann et al., 2023) and combine litters (Kim et al., 2011). While keeping a larger number of animals post-weaning (or preserving the whole litter together; Kim et al., 2011; McCallum et al., 2010; Yap & Richardson, 2007; Zimmermann et al., 2023) would maintain consistency with pre-weaning conditions to minimize the stress of weaning, this may not be sustainable at older ages given the size of the animals and local animal care guidelines.
EXPERIMENTAL DESIGN
Given the developmental constraints delineated above, it seems likely that differences in experimental design, a known source of variability, may disproportionally affect developmental fear studies. A major concern when conducting fear studies across development is the possibility that animals of different ages may experience pain and respond to foot shocks differently, thereby influencing their fear responses. To account for that possibility, some labs vary their fear conditioning protocols according to animal age to obtain equivalent freezing levels at fear retrieval across groups, most often by increasing CS-US pairings for younger mice (Gogolla et al., 2009; Samifanni et al., 2021) and rats (Brown et al., 2021; Ganella et al., 2016; Kim et al., 2009; Kim & Richardson, 2007a, 2008). While this addresses one important variable, titrating the experimental design to normalize freezing output may overlook impacts of fear conditioning outside of freezing. Conversely, the use of a single training protocol across ages (Akers et al., 2012; Koppensteiner et al., 2019; Li et al., 2018; Park et al., 2017a; Park, Ganella, & Kim, 2020; Pattwell et al., 2012; Raineki et al., 2010; Samifanni et al., 2021) might discount age-specific impacts of stress and pain that could affect other aspects of emotional learning beyond the standard metrics analyzed in fear experiments. As is the norm for developmental studies (and beyond), any experimental design choice comes with intrinsic limitations that should be taken into account when interpreting results. Measuring a broader range of behavioral output metrics, including grooming, darting, jumping, and rearing, may help with validation of the choice of experimental design, especially given reports of sex differences in some of these metrics (Borkar et al., 2020; Colom-Lapetina et al., 2019; Gruene et al., 2015; Mitchell et al., 2022; Seemiller et al., 2021; Shansky, 2018).
Due to the increased contextual generalization seen in infant and juvenile mice (Ramsaran et al., 2023), great consideration should be given to the elements used to distinguish between contexts at early ages, especially when analyzing auditory fear. Auditory fear training often occurs in a context that is ideally distinguishable from the fear retrieval context (where the animal is exposed to the CS only) through the use of olfactory (e.g., distinct neutral odors), visual (e.g., different color or patterned panel inserts), or tactile (e.g., metal versus plastic floors) cues. Achieving successful discrimination between contexts is important to avoid compounding freezing to the CS with any residual generalized freezing to the training context. To determine whether the degree of dissimilarity between contexts is sufficient to overcome age-dependent differences in contextual generalization in auditory fear experiments, it is important to track baseline freezing data in the fear retrieval (alternative) context prior to tone onset across the ages tested and adjust experimental parameters to achieve equivalent discrimination, if necessary. Early optimization of contextual parameters and transparent reporting of baseline freezing in auditory fear conditioning experiments using young rodents would improve rigor and data reliability.
Another way in which experimental design affects developmental fear pertains to the study of adolescent extinction. While a considerable literature reports an extinction learning and/or retention deficit in adolescence, this is not always replicated (for a more extensive discussion, see Bisby et al., 2021; for an integration of their parameters and others highlighted here, see Supporting Information Table S1). One factor that may contribute to inconsistency in the reports of adolescent extinction learning is experimental design. Extinction protocols vary by the number of CS presentations per trial and the number of extinction trials per animal (e.g., single extinction trial versus multiple trials across consecutive days; see Supporting Information Table S1). Importantly, Kim et al. (2011) and McCallum et al. (2010) found that doubling the extinction protocol rescued the adolescent extinction deficit. This is consistent with another study conducted by Gerhard and Meyer (2021) that saw no extinction deficit in adolescent mice, but used a greater number of tone presentations compared to studies that do see a deficit (i.e., 40 vs. 20). It is important to mention that differences in the design of extinction protocols also impact adult rodents (Cain et al., 2003; Gerhard & Meyer, 2021; Ledgerwood et al., 2005; MacPherson et al., 2013), which demonstrate greater extinction retention when trials are spaced across several days rather than in a single day (in mice: Cain et al., 2003; Gerhard & Meyer, 2021). Thus, it is possible that conducting extinction trials across multiple days with massed trials may facilitate extinction learning in both younger and older rodents. Taken together, the choice of extinction protocol appears to be a major determinant of whether a deficit in extinction will be detected in adolescent rodents. Importantly, while this conclusion does not preclude the existence of a higher threshold for successful fear extinction in adolescent rodents compared to adults, it might inform experimental design choices when conducting extinction experiments with adolescent animals.
Overall, several factors need to be considered when designing and interpreting developmental fear experiments. Although experimental designs vary in many other ways not covered here (e.g., intertrial intervals, pre-exposure to context, handling), it is clear that choices made when designing fear experiments may have a greater impact when studying development and should be thoroughly validated internally and carefully guided by existing literature.
BEHAVIORAL ANALYSIS
Historically, rodent freezing was quantified predominantly through manual scoring (Bourtchuladze et al., 1994; Fanselow & Bolles, 1979; Paylor et al., 1994; Phillips & LeDoux, 1992). This was followed by a substantial shift toward computer-assisted systems for automated scoring (Anagnostaras et al., 2000), whose sensitivity has substantially improved in recent years. Even so, the size of infant mice poses considerable challenges for quantifying fear behavior in early life. Some features of young mice (e.g., ears and paws) are less easily distinguishable on camera due to their small size, and the animals may at times be partially or completely occluded from view. This is a serious limitation of certain fear chamber setups, whereby the protruding lip of the grid floor nearest to the camera can partially or even completely block the view of juvenile or infant mice. These developmental constraints may explain why manual scoring remains the method of choice for many developmental fear researchers.
The majority of studies analyzing rodent fear behavior in development have a blinded experimenter manually score freezing across the whole behavioral session (Carew & Rudy, 1991; Colon et al., 2018; Glavonic et al., 2023; Pattwell et al., 2012; Revillo et al., 2016; Revillo, Paglini, & Arias, et al., 2014). As this can be quite labor-intensive, a subset of studies uses a time-sampling approach, in which a binary freezing/no freezing category is assigned to a particular time interval of 3 s (Baker & Richardson, 2015; Bisby et al., 2018; Harmon-Jones & Richardson, 2021; Kim et al., 2009, 2011; Kim & Richardson, 2007a,b, 2008; McCallum et al., 2010; Yap & Richardson, 2007; Zimmermann et al., 2023), 5 s (Cain et al., 2003; Hefner & Holmes, 2007), or 10 s (Kutlu et al., 2018; Morgan & Pfaff, 2001). Although manual scoring is time-consuming and may carry the possibility of observer bias, it allows for quantification of complex behaviors that might be difficult to score automatically (such as grooming and rearing, as in Colon et al., 2018, but see below). Manual scoring also minimizes data loss in cases of partial visual occlusion of the animal, since a human observer may be still able to assess freezing in those instances. However, manual analysis does not allow for easy or consistent adjustment in the parameters for measuring freezing (e.g., minimum freezing threshold, or how long the animal must freeze in order for it to count as one freezing instance), making direct comparisons with automated metrics challenging.
Video-assisted scoring of freezing behavior is a very useful tool, as it dramatically reduces the time required to score behavioral data and removes observer bias. The most common automated freezing scoring software programs used in developmental fear studies are VideoFreeze (MedAssociates) (Ferrara et al., 2020; Ganella et al., 2017, 2018; Gerhard & Meyer, 2021; Lanjewar et al., 2023; McDermott et al., 2012; Park et al., 2020; Perry et al., 2020), FreezeFrame (Actimetrics) (Akers et al., 2012; Ishii et al., 2019; Koppensteiner et al., 2019; Riddle et al., 2013), and Ethovision (Noldus, more commonly used for non-freezing behaviors) (Bailoo et al., 2020; Bath et al., 2016). See Table 1 for a comparison of freezing quantification methods within the parameters discussed here. These software tools provide control over parameters used to optimize freezing quantification, such as the motion threshold, sample rate, and minimum freeze duration. Critically, due to the factors mentioned above, the freezing parameters used to measure adult behavior may not optimally capture freezing in younger animals. Thus, when using this method in developmental studies, it is important to first optimize freezing parameters across the ages tested and to maintain consistency within and between labs.
Quantitative methods | Time investment | Motion threshold, minimum freeze duration | Visual occlusion |
---|---|---|---|
Manual scoring | ↑↑↑ | X | ✓ |
VideoFreeze | ↑ | ✓ | X |
FreezeFrame | ↑ | ✓b | X |
Ethovision | ↑ | ✓ | X |
DeepLabCut | ↑↑c | ✓c | ? |
- a Summary of methods and analysis software used to quantify freezing in young rodents. Time investment refers to amount of time required to score freezing. Motion threshold and minimum freeze duration depicts which methods offer the ability to manipulate these parameters. Visual occlusion refers to whether each quantitative method can bypass the issue of incorrect scoring of freezing when young rodents are partially occluded in the fear apparatus.
- b The motion threshold for FreezeFrame is adjusted for each individual animal.
- c DeepLabCut (DLC) may require more time investment to initially train the system, but is less time-consuming afterwards. It is possible to set motion threshold, minimum freeze duration, and other parameters using a custom code to analyze the DLC output.
Typically, studies vary motion thresholds to capture freezing across species and ages. As a reference, developmental fear studies in mice often use the lowest VideoFreeze motion threshold of 18 arbitrary units (au) (Gerhard & Meyer, 2021; Lanjewar et al., 2023; Samifanni et al., 2021), while studies in rats use from 50 au (Ganella et al., 2017 at P34; Handford et al., 2014 in adults; Perry et al., 2020 at P35) to 70 au (Park et al., 2017, 2020 at P18). Some studies use different VideoFreeze motion threshold values for each age within the same study (i.e., one threshold value is used for juveniles while another is used for adults) in order to best correlate with manual scoring conducted on a subset of animals (Ganella et al., 2016, 2018). Motion threshold values are rarely reported for FreezeFrame, likely because this metric is adjusted per individual animal within the software.
In addition to the motion threshold, the choice of minimum duration required to constitute a freezing episode significantly impacts behavioral output and consequent interpretation. While many studies do not publish this value, several reported the use of 1 s (Lanjewar et al., 2023; Perry et al., 2020; Samifanni et al., 2021) or 2 s (Chocyk et al., 2014). Harmonization of these metrics across labs may reduce variability and improve convergence between studies.
Finally, the increased sensitivity of recent behavioral tracking methods such as DeepLabCut (Mathis et al., 2018) may help address some of the developmental constraints in automated behavior tracking. As these methods can detect and track smaller body parts (i.e., tails and ears) of young animals, they should be tested and validated in the case of visual occlusion. Tools such as DeepLabCut are becoming standard in behavioral neuroscience and can successfully capture a wide range of behavioral parameters in young mice (Gerhard et al., 2023), carrying great potential for reliable and diverse quantification of developmental fear behavior.
CONCLUSION
Developmental studies offer unique insight into how brain circuits mature to support behavior, but also carry unique challenges and sources of variability. The aim of this overview is to identify and discuss factors that may contribute to variability across developmental fear studies in mice and offer recommendations aimed at harmonizing practices across labs. Overall, transparency in the reporting of experimental parameters—including how animals are bred, the type of bedding used, when and how animals are weaned, the experimental design, and how fear behavior is analyzed—is strongly encouraged so that these variables can be weighed into the interpretation of findings across studies. The successful adaptation of the use of viral, opto/chemogenetic, and calcium imaging tools in young rodents has significantly expanded the depth and breadth of inquiry into the neural mechanisms of developmental fear processes. Harmonization of experimental procedures across labs will facilitate consensus and increase cohesion in this fast-expanding research field.
Acknowledgments
This work was supported by grants from the Natural Sciences and Engineering Research Council of Canada (NSERC CGS D – 569380 – 2022 to JW and RGPIN-2017-06344 to MAC), an Ontario Graduate Scholarship (OGS 2023 to HP), and grants to MAC from the Canadian Institutes of Health Research (CIHR; PJT 399790), the Human Frontier Science Program Organization (CDA00009/2018 and RGY0072/2019), and the SickKids Foundation and CIHR–Institute of Human Development, Child and Youth Health (NI19-1132R).
Author Contributions
Hanista Premachandran : Conceptualization; writing—original draft; writing—review and editing. Jennifer Wilkin : Conceptualization; writing—original draft; writing—review and editing. Maithe Arruda-Carvalho : Conceptualization; funding acquisition; supervision; writing—review and editing.
Conflict of Interest
The authors have no conflict of interest to disclose.
Open Research
Data Availability Statement
Data sharing not applicable to this article as no datasets were generated or analyzed during the current study
Supporting Information
Filename | Description |
---|---|
cpz11040-sup-0001-SuppMat.docx1.2 MB | Table S1 rodent study comparison.doc A comparison of experimental designs for auditory fear extinction in adolescent mice and rats |
Please note: The publisher is not responsible for the content or functionality of any supporting information supplied by the authors. Any queries (other than missing content) should be directed to the corresponding author for the article.
Literature Cited
- Akers, K. G., Arruda-Carvalho, M., Josselyn, S. A., & Frankland, P. W. (2012). Ontogeny of contextual fear memory formation, specificity, and persistence in mice. Learning and Memory , 19(12), 598–604. https://doi.org/10.1101/lm.027581.112
- Akers, K. G., Martinez-Canabal, A., Restivo, L., Yiu, A. P., de Cristofaro, A., Hsiang, H. L., Wheeler, A. L., Guskjolen, A., Niibori, Y., Shoji, H., Ohira, K., Richards, B. A., Miyakawa, T., Josselyn, S. A., & Frankland, P. W. (2014). Hippocampal neurogenesis regulates forgetting during adulthood and infancy. Science , 344(6184), 598–602. https://doi.org/10.1126/science.1248903
- Akirav, I., & Maroun, M. (2007). The role of the medial prefrontal cortex-amygdala circuit in stress effects on the extinction of fear. Neural Plasticity , 2007, 030873. https://doi.org/10.1155/2007/30873
- Alberini, C. M., & Travaglia, A. (2017). Infantile amnesia: A critical period of learning to learn and remember. Journal of Neuroscience , 37(24), 5783–5795. https://doi.org/10.1523/JNEUROSCI.0324-17.2017
- An, B., Kim, J., Park, K., Lee, S., Song, S., & Choi, S. (2017). Amount of fear extinction changes its underlying mechanisms. eLife , 6, e25224. https://doi.org/10.7554/eLife.25224
- Anagnostaras, S. G., Josselyn, S. A., Frankland, P. W., & Silva, A. J. (2000). Computer-assisted behavioral assessment of Pavlovian fear conditioning in mice. Learning and Memory , 7(1), 58–72. https://doi.org/10.1101/lm.7.1.58
- Arnsten, A. F. T. (2009). Stress signalling pathways that impair prefrontal cortex structure and function. Nature Reviews Neuroscience , 10(6), 410–422. https://doi.org/10.1038/nrn2648
- Arruda-Carvalho, M., & Clem, R. L. (2014). Pathway-selective adjustment of prefrontal-amygdala transmission during fear encoding. Journal of Neuroscience , 34(47), 15601–15609. https://doi.org/10.1523/JNEUROSCI.2664-14.2014
- Arruda-Carvalho, M., & Clem, R. L. (2015). Prefrontal-amygdala fear networks come into focus. Frontiers in Systems Neuroscience , 9, 145. https://doi.org/10.3389/fnsys.2015.00145
- Arruda-Carvalho, M., Wu, W. C., Cummings, K. A., & Clem, R. L. (2017). Optogenetic examination of prefrontal-amygdala synaptic development. Journal of Neuroscience , 37(11), 2976–2985. https://doi.org/10.1523/JNEUROSCI.3097-16.2017
- Bailoo, J. D., Murphy, E., Varholick, J. A., Novak, J., Palme, R., & Würbel, H. (2018). Evaluation of the effects of space allowance on measures of animal welfare in laboratory mice. Scientific Reports , 8(1), 1–11. https://doi.org/10.1038/s41598-017-18493-6
- Bailoo, J. D., Voelkl, B., Varholick, J., Novak, J., Murphy, E., Rosso, M., Palme, R., & Würbel, H. (2020). Effects of weaning age and housing conditions on phenotypic differences in mice. Scientific Reports , 10(1), 1–13. https://doi.org/10.1038/s41598-020-68549-3
- Baker, K. D., Gray, A. R., & Richardson, R. (2017). The development of perineuronal nets around parvalbumin GABAergic neurons in the medial prefrontal cortex and basolateral amygdala of rats. Behavioral Neuroscience , 131(4), 289–303. https://doi.org/10.1037/bne0000203
- Baker, K. D., McNally, G. P., & Richardson, R. (2013). Memory retrieval before or after extinction reduces recovery of fear in adolescent rats. Learning and Memory , 20(9), 467–473. https://doi.org/10.1101/lm.031989.113
- Baker, K. D., McNally, G. P., & Richardson, R. (2018). d-Cycloserine facilitates fear extinction in adolescent rats and differentially affects medial and lateral prefrontal cortex activation. Progress in Neuro-Psychopharmacology and Biological Psychiatry , 86, 262–269. https://doi.org/10.1016/j.pnpbp.2018.06.007
- Baker, K. D., & Richardson, R. (2015). Forming competing fear learning and extinction memories in adolescence makes fear difficult to inhibit. Learning and Memory , 22(11), 537–543. https://doi.org/10.1101/lm.039487.114
- Baker, K. D., & Richardson, R. (2017). Pharmacological evidence that a failure to recruit NMDA receptors contributes to impaired fear extinction retention in adolescent rats. Neurobiology of Learning and Memory , 143, 18–26. https://doi.org/10.1016/j.nlm.2016.10.014
- Baker-Andresen, D., Flavell, C. R., Li, X., & Bredy, T. W. (2013). Activation of BDNF signaling prevents the return of fear in female mice. Learning and Memory , 20(5), 237–240. https://doi.org/10.1101/lm.029520.112
- Balogh, S. A., & Wehner, J. M. (2003). Inbred mouse strain differences in the establishment of long-term fear memory. Behavioural Brain Research , 140(1–2), 97–106. https://doi.org/10.1016/S0166-4328(02)00279-6
- Barbayannis, G., Franco, D., Wong, S., Galdamez, J., Romeo, R. D., & Bauer, E. P. (2017). Differential effects of stress on fear learning and activation of the amygdala in pre-adolescent and adult male rats. Neuroscience , 360, 210–219. https://doi.org/10.1016/j.neuroscience.2017.07.058
- Bath, K. G., Manzano-Nieves, G., & Goodwill, H. (2016). Early life stress accelerates behavioral and neural maturation of the hippocampus in male mice. Hormones and Behavior , 82, 64–71. https://doi.org/10.1016/j.yhbeh.2016.04.010
- Bechard, A., & Mason, G. (2010). Leaving home: A study of laboratory mouse pup independence. Applied Animal Behaviour Science , 125(3–4), 181–188. https://doi.org/10.1016/j.applanim.2010.04.006
- Bell, M. R. (2018). Comparing postnatal development of gonadal hormones and associated social behaviors in rats, mice, and humans. Endocrinology , 159(7), 2596–2613. https://doi.org/10.1210/en.2018-00220
- Berdoy, M., & Drickamer, L. C. (2007). Comparative social organization and life history of Rattus and Mus. In J. O. Wolff & P. W. Sherman (Eds.) Rodent Societies: An Ecological & Evolutionary Perspective (pp. 380–392). University of Chicago Press.
- Berry, A., Bellisario, V., Capoccia, S., Tirassa, P., Calza, A., Alleva, E., & Cirulli, F. (2012). Social deprivation stress is a triggering factor for the emergence of anxiety- and depression-like behaviours and leads to reduced brain BDNF levels in C57BL/6J mice. Psychoneuroendocrinology , 37(6), 762–772. https://doi.org/10.1016/j.psyneuen.2011.09.007
- Bessières, B., Jia, M., Travaglia, A., & Alberini, C. M. (2019). Developmental changes in plasticity, synaptic, glia, and connectivity protein levels in rat basolateral amygdala. Learning and Memory , 26(11), 436–448. https://doi.org/10.1101/lm.049866.119
- Bisby, M. A., Baker, K. D., & Richardson, R. (2018). Elucidating the mechanisms of fear extinction in developing animals: A special case of NMDA receptor-independent extinction in adolescent rats. Learning and Memory , 25(4), 158–164. https://doi.org/10.1101/lm.047209.117
- Bisby, M. A., Baker, K. D., & Richardson, R. (2020). Deficits in opioid receptor-mediated prediction error contribute to impaired fear extinction during adolescence. Behaviour Research and Therapy , 133, 103713. https://doi.org/10.1016/j.brat.2020.103713
- Bisby, M. A., Richardson, R., & Baker, K. D. (2020). Developmental differences in the effects of CB1/2R agonist WIN55212-2 on extinction of learned fear. Progress in Neuro-Psychopharmacology and Biological Psychiatry , 99, 109834. https://doi.org/10.1016/j.pnpbp.2019.109834
- Bisby, M. A., Stylianakis, A. A., Baker, K. D., & Richardson, R. (2021). Fear extinction learning and retention during adolescence in rats and mice: A systematic review. Neuroscience and Biobehavioral Reviews , 131, 1264–1274. https://doi.org/10.1016/j.neubiorev.2021.10.044
- Blair, M. G., Nguyen, N. N. Q., Albani, S. H., L'Etoile, M. M., Andrawis, M. M., Owen, L. M., Oliveira, R. F., Johnson, M. W., Purvis, D. L., Sanders, E. M., Stoneham, E. T., Xu, H., & Dumas, T. C. (2013). Developmental changes in structural and functional properties of hippocampal AMPARs parallels the emergence of deliberative spatial navigation in juvenile rats. Journal of Neuroscience , 33(30), 12218–12228. https://doi.org/10.1523/JNEUROSCI.4827-12.2013
- Blanchard, R. J., & Blanchard, D. C. (1969). Crouching as an index of fear. Journal of Comparative and Physiological Psychology , 67(3), 370–375. https://doi.org/10.1037/h0026779
- Bolles, R. C., & Woods, P. J. (1964). The ontogeny of behaviour in the albino rat. Animal Behaviour , 12(4), 427–441. https://doi.org/10.1016/0003-3472(64)90062-4
- Borkar, C. D., Dorofeikova, M., Le, Q. S. E., Vutukuri, R., Vo, C., Hereford, D., Resendez, A., Basavanhalli, S., Sifnugel, N., & Fadok, J. P. (2020). Sex differences in behavioral responses during a conditioned flight paradigm. Behavioural Brain Research , 389, 112623. https://doi.org/10.1016/j.bbr.2020.112623
- Bosch, D., & Ehrlich, I. (2015). Postnatal maturation of GABAergic modulation of sensory inputs onto lateral amygdala principal neurons. Journal of Physiology , 593(19), 4387–4409. https://doi.org/10.1113/JP270645
- Bourtchuladze, R., Frenguelli, B., Blendy, J., Cioffi, D., Schutz, G., & Silva, A. J. (1994). Deficient long-term memory in mice with a targeted mutation of the cAMP-responsive element-binding protein. Cell , 79(1), 59–68. https://doi.org/10.1016/0092-8674(94)90400-6
- Bouton, M. E., & Bolles, R. C. (1979a). Contextual control of the extinction of conditioned fear. Learning and Motivation , 10(4), 248–265. https://doi.org/10.1016/0023-9690(79)90057-2
- Bouton, M. E., & Bolles, R. C. (1979b). Role of conditioned contextual stimuli in reinstatement of extinguished fear. Journal of Experimental Psychology: Animal Behavior Processes , 5(4), 368–378. https://doi.org/10.1037/0097-7403.5.4.368
- Brasser, S. M., & Spear, N. E. (2004). Contextual conditioning in infants, but not older animals, is facilitated by CS conditioning. Neurobiology of Learning and Memory , 81(1), 46–59. https://doi.org/10.1016/S1074-7427(03)00068-6
- Brenhouse, H. C., & Andersen, S. L. (2011). Developmental trajectories during adolescence in males and females: A cross-species understanding of underlying brain changes. Neuroscience and Biobehavioral Reviews , 35(8), 1687–1703. https://doi.org/10.1016/j.neubiorev.2011.04.013
- Brown, K. L., Sodoma, M. J., & Freeman, J. H. (2021). Factors influencing developmental differences in retention of Pavlovian fear conditioning. Behavioral Neuroscience , 135(4), 498–517. https://doi.org/10.1037/bne0000446
- Brust, V., Schindler, P. M., & Lewejohann, L. (2015). Lifetime development of behavioural phenotype in the house mouse (Mus musculus). Frontiers in Zoology , 12(1), 17. https://doi.org/10.1186/1742-9994-12-S1-S17
- Bryant, C. D., Zhang, N. N., Sokoloff, G., Fanselow, M. S., Ennes, H. S., Palmer, A. A., & McRoberts, J. A. (2008). Behavioral differences among C57BL/6 substrains: Implications for transgenic and knockout studies. Journal of Neurogenetics , 22(4), 315–331. https://doi.org/10.1080/01677060802357388
- Bukalo, O., Pinard, C. R., Silverstein, S., Brehm, C., Hartley, N. D., Whittle, N., Colacicco, G., Busch, E., Patel, S., Singewald, N., & Holmes, A. (2015). Prefrontal inputs to the amygdala instruct fear extinction memory formation. Science Advances , 1(6), 1–8. https://doi.org/10.1126/sciadv.1500251
- Burgos-Robles, A., Vidal-Gonzalez, I., & Quirk, G. J. (2009). Sustained conditioned responses in prelimbic prefrontal neurons are correlated with fear expression and extinction failure. Journal of Neuroscience , 29(26), 8474–8482. https://doi.org/10.1523/JNEUROSCI.0378-09.2009
- Caballero, A., Granberg, R., & Tseng, K. Y. (2016). Mechanisms contributing to prefrontal cortex maturation during adolescence. Neuroscience and Biobehavioral Reviews , 70, 4–12. https://doi.org/10.1016/j.neubiorev.2016.05.013
- Caballero, A., & Tseng, K. Y. (2016). GABAergic function as a limiting factor for prefrontal maturation during adolescence. Trends in Neurosciences , 39(7), 441–448. https://doi.org/10.1016/j.tins.2016.04.010
- Cain, C. K., Blouin, A. M., & Barad, M. (2003). Temporally massed CS presentations generate more fear extinction than spaced presentations. Journal of Experimental Psychology: Animal Behavior Processes , 29(4), 323–333. https://doi.org/10.1037/0097-7403.29.4.323
- Callaghan, B. L., Li, S., & Richardson, R. (2014). The elusive engram: What can infantile amnesia tell us about memory? Trends in Neurosciences , 37(1), 47–53. https://doi.org/10.1016/j.tins.2013.10.007
- Callaghan, B. L., & Richardson, R. (2011). Maternal separation results in early emergence of adult-like fear and extinction learning in infant rats. Behavioral Neuroscience , 125(1), 20–28. https://doi.org/10.1037/a0022008
- Callaghan, B. L., & Richardson, R. (2012a). Early-life stress affects extinction during critical periods of development: An analysis of the effects of maternal separation on extinction in adolescent rats. Stress , 15(6), 671–679. https://doi.org/10.3109/10253890.2012.667463
- Callaghan, B. L., & Richardson, R. (2012b). The effect of adverse rearing environments on persistent memories in young rats: Removing the brakes on infant fear memories. Translational Psychiatry , 2(7), e138. https://doi.org/10.1038/tp.2012.65
- Callaghan, B. L., & Richardson, R. (2013). Early experiences and the development of emotional learning systems in rats. Biology of Mood and Anxiety Disorders , 3(1), 8. https://doi.org/10.1186/2045-5380-3-8
- Callaghan, B. L., & Richardson, R. (2014). Early emergence of adult-like fear renewal in the developing rat after chronic corticosterone treatment of the dam or the pups. Behavioral Neuroscience , 128(5), 594–602. https://doi.org/10.1037/bne0000009
- Callaghan, B. L., & Tottenham, N. (2016). The stress acceleration hypothesis: Effects of early-life adversity on emotion circuits and behavior. Current Opinion in Behavioral Sciences , 7, 76–81. https://doi.org/10.1016/j.cobeha.2015.11.018
- Camp, M. C., MacPherson, K. P., Lederle, L., Graybeal, C., Gaburro, S., Debrouse, L. M., Ihne, J. L., Bravo, J. A., O'Connor, R. M., Ciocchi, S., Wellman, C. L., Lüthi, A., Cryan, J. F., Singewald, N., & Holmes, A. (2012). Genetic strain differences in learned fear inhibition associated with variation in neuroendocrine, autonomic, and amygdala dendritic phenotypes. Neuropsychopharmacology , 37(6), 1534–1547. https://doi.org/10.1038/npp.2011.340
- Campbell, B. A., & Ampuero, M. X. (1985). Dissociation of autonomic and behavioral components of conditioned fear during development in the rat. Behavioral Neuroscience , 99(6), 1089–1102. https://doi.org/10.1037/0735-7044.99.6.1089
- Campbell, B. A., & Campbell, E. H. (1962). Retention and extinction of learned fear in infant and adult rats. Journal of Comparative and Physiological Psychology , 55(1), 1–8. https://doi.org/10.1037/h0049182
- Campbell, B. A., & Spear, N. E. (1972). Ontogeny of memory. Psychological Review , 79(3), 215–236. https://doi.org/10.1037/h0032690
- Canadian Council on Animal Care (CCAC). (2022). CCAC guidelines: Mice: Frequently Asked Questions. Revision: September, 2022.
- Carew, M. B., & Rudy, J. W. (1991). Multiple functions of context during conditioning: A developmental analysis. Developmental Psychobiology , 24(3), 191–209. https://doi.org/10.1002/dev.420240305
- Casey, B. J., Pattwell, S. S., Glatt, C. E., & Lee, F. S. (2013). Treating the developing brain: Implications from human imaging and mouse genetics. Annual Review of Medicine , 64, 427–439. https://doi.org/10.1146/annurev-med-052611-130408
- Cazares, V. A., Rodriguez, G., Parent, R., Ouillette, L., Glanowska, K. M., Moore, S. J., & Murphy, G. G. (2019). Environmental variables that ameliorate extinction learning deficits in the 129S1/SvlmJ mouse strain. Genes, Brain and Behavior , 18(7), e12575. https://doi.org/10.1111/gbb.12575
- Chang, Y. J., Yang, C. H., Liang, Y. C., Yeh, C. M., Huang, C. C., & Hsu, K. S. (2009). Estrogen modulates sexually dimorphic contextual fear extinction in rats through estrogen receptor β. Hippocampus , 19(11), 1142–1150. https://doi.org/10.1002/hipo.20581
- Chen, C. V., Chaby, L. E., Nazeer, S., & Liberzon, I. (2018). Effects of trauma in adulthood and adolescence on fear extinction and extinction retention: Advancing animal models of posttraumatic stress disorder. Frontiers in Behavioral Neuroscience , 12, 247. https://doi.org/10.3389/fnbeh.2018.00247
- Cho, J. H., Deisseroth, K., & Bolshakov, V. Y. (2013). Synaptic encoding of fear extinction in mPFC-amygdala circuits. Neuron , 80(6), 1491–1507. https://doi.org/10.1016/j.neuron.2013.09.025
- Chocyk, A., Przyborowska, A., Makuch, W., Majcher-Maślanka, I., Dudys, D., & Wedzony, K. (2014). The effects of early-life adversity on fear memories in adolescent rats and their persistence into adulthood. Behavioural Brain Research , 264, 161–172. https://doi.org/10.1016/j.bbr.2014.01.040
- Cohen, M. M., Jing, D., Yang, R. R., Tottenham, N., Lee, F. S., & Casey, B. J. (2013). Early-life stress has persistent effects on amygdala function and development in mice and humans. Proceedings of the National Academy of Sciences of the United States of America , 110(45), 18274–18278. https://doi.org/10.1073/pnas.1310163110
- Colom-Lapetina, J., Li, A. J., Pelegrina-Perez, T. C., & Shansky, R. M. (2019). Behavioral diversity across classic rodent models is sex-dependent. Frontiers in Behavioral Neuroscience , 13, 45. https://doi.org/10.3389/fnbeh.2019.00045
- Colon, L., Odynocki, N., Santarelli, A., & Poulos, A. M. (2018). Sexual differentiation of contextual fear responses. Learning and Memory , 25(5), 230–240. https://doi.org/10.1101/lm.047159.117
- Colón, L., Peru, E., Zuloaga, D. G., & Poulos, A. M. (2023). Contributions of gonadal hormones in the sex-specific organization of context fear learning. PLoS ONE , 18(3), e0282293. https://doi.org/10.1371/journal.pone.0282293
- Corcoran, K. A., & Maren, S. (2004). Factors regulating the effects of hippocampal inactivation on renewal of conditional fear after extinction. Learning and Memory , 11(5), 598–603. https://doi.org/10.1101/lm.78704
- Corcoran, K. A., & Quirk, G. J. (2007). Activity in prelimbic cortex is necessary for the expression of learned, but not innate, fears. Journal of Neuroscience , 27(4), 840–844. https://doi.org/10.1523/JNEUROSCI.5327-06.2007
- Cotella, E. M., Gómez, A. S., Lemen, P., Chen, C., Fernández, G., Hansen, C., Herman, J. P., & Paglini, M. G. (2019). Long-term impact of chronic variable stress in adolescence versus adulthood. Progress in Neuro-Psychopharmacology and Biological Psychiatry , 88, 303–310. https://doi.org/10.1016/j.pnpbp.2018.08.003
- Cousens, G., & Otto, T. (1998). Both pre- and posttraining excitotoxic lesions of the basolateral amygdala abolish the expression of olfactory and contextual fear conditioning. Behavioral Neuroscience , 112(5), 1092–1103. https://doi.org/10.1037/0735-7044.112.5.1092
- Cowan, C. S. M., Callaghan, B. L., & Richardson, R. (2013). Acute early-life stress results in premature emergence of adult-like fear retention and extinction relapse in infant rats. Behavioral Neuroscience , 127(5), 703–711. https://doi.org/10.1037/a0034118
- Cowan, C. S. M., & Richardson, R. (2018). A brief guide to studying fear in developing rodents: Important considerations and common pitfalls. Current Protocols in Neuroscience , 83(1), e44. https://doi.org/10.1002/cpns.44
- Crestani, A. P., Lotz, F. N., Casagrande, M. A., Popik, B., Guerra, K. T. K., de Oliveira Alvares, L., & Quillfeldt, J. A. (2022). Adolescent female rats undergo full systems consolidation of an aversive memory, while males of the same age fail to discriminate contexts. Behavioral Neuroscience , 136(2), 172–181. https://doi.org/10.1037/bne0000501
- Cruz, E., López, A. V., & Porter, J. T. (2014). Spontaneous recovery of fear reverses extinction-induced excitability of infralimbic neurons. PLoS ONE , 9(8), e103596. https://doi.org/10.1371/journal.pone.0103596
- Cruz, E., Soler-Cedenõ, O., NegrÓn, G., Criado-Marrero, M., ChomprÁ, G., & Porter, J. T. (2015). Infralimbic ephb2 modulates fear extinction in adolescent rats. Journal of Neuroscience , 35(36). https://doi.org/10.1523/JNEUROSCI.4254-14.2015
- Curley, J. P., Jordan, E. R., Swaney, W. T., Izraelit, A., Kammel, S., & Champagne, F. A. (2009). The meaning of weaning: Influence of the weaning period on behavioral development in mice. Developmental Neuroscience , 31(4), 318–331. https://doi.org/10.1159/000216543
- Debiec, J., LeDoux, J. E., & Nader, K. (2002). Cellular and systems reconsolidation in the hippocampus. Neuron , 36(3), 527–538. https://doi.org/10.1016/S0896-6273(02)01001-2
- Den, M. L., Altmann, S. R., & Richardson, R. (2014). Comparison of the short- and long-term effects of corticosterone exposure on extinction in adolescence versus adulthood. Behavioral Neuroscience , 128(6), 722–735. https://doi.org/10.1037/bne0000022
- Do-Monte, F. H., Manzano-Nieves, G., Quiñones-Laracuente, K., Ramos-Medina, L., & Quirk, G. J. (2015). Revisiting the role of infralimbic cortex in fear extinction with optogenetics. Journal of Neuroscience , 35(8), 3607–3615. https://doi.org/10.1523/JNEUROSCI.3137-14.2015
- Donato, F., Alberini, C. M., Amso, D., Dragoi, G., Dranovsky, A., & Newcombe, N. S. (2021). The ontogeny of hippocampus-dependent memories. Journal of Neuroscience , 41(5), 920–926. https://doi.org/10.1523/JNEUROSCI.1651-20.2020
- dos Santos Oliveira, L., da Silva, L. P., da Silva, A. I., Magalhães, C. P., de Souza, S. L., & de Castro, R. M. (2011). Effects of early weaning on the circadian rhythm and behavioral satiety sequence in rats. Behavioural Processes , 86(1), 119–124. https://doi.org/10.1016/j.beproc.2010.10.001
- Drummond, K. D., Waring, M. L., Faulkner, G. J., Blewitt, M. E., Perry, C. J., & Kim, J. H. (2021). Hippocampal neurogenesis mediates sex-specific effects of social isolation and exercise on fear extinction in adolescence. Neurobiology of Stress , 15, 100367. https://doi.org/10.1016/j.ynstr.2021.100367
- Duvarci, S., & Pare, D. (2014). Amygdala microcircuits controlling learned fear. Neuron , 82(5), 966–980. https://doi.org/10.1016/j.neuron.2014.04.042
- Ehrlich, I., Humeau, Y., Grenier, F., Ciocchi, S., Herry, C., & Lüthi, A. (2009). Amygdala inhibitory circuits and the control of fear memory. Neuron , 62(6), 757–771. https://doi.org/10.1016/j.neuron.2009.05.026
- Eltokhi, A., Kurpiers, B., & Pitzer, C. (2020). Behavioral tests assessing neuropsychiatric phenotypes in adolescent mice reveal strain- and sex-specific effects. Scientific Reports , 10(1), 11263. https://doi.org/10.1038/s41598-020-67758-0
- Fanselow, M. S. (1980). Conditional and unconditional components of post-shock freezing. The Pavlovian Journal of Biological Science , 15(4), 177–182. https://doi.org/10.1007/BF03001163
- Fanselow, M. S. (2000). Contextual fear, gestalt memories, and the hippocampus. Behavioural Brain Research , 110(1–2), 73–81. https://doi.org/10.1016/S0166-4328(99)00186-2
- Fanselow, M. S., & Bolles, R. C. (1979). Naloxone and shock-elicited freezing in the rat. Journal of Comparative and Physiological Psychology , 93(4), 736–744. https://doi.org/10.1037/h0077609
- Ferrara, N. C., Mrackova, E., Loh, M. K., Padival, M., & Rosenkranz, J. A. (2020). Fear learning enhances prefrontal cortical suppression of auditory thalamic inputs to the amygdala in adults, but not adolescents. International Journal of Molecular Sciences , 21(8), 3008. https://doi.org/10.3390/ijms21083008
- Ferrara, N. C., Trask, S., & Rosenkranz, J. A. (2021). Maturation of amygdala inputs regulate shifts in social and fear behaviors: A substrate for developmental effects of stress. Neuroscience and Biobehavioral Reviews , 125, 11–25. https://doi.org/10.1016/j.neubiorev.2021.01.021
- Ganella, D. E., & Kim, J. H. Y. (2014). Developmental rodent models of fear and anxiety: From neurobiology to pharmacology. British Journal of Pharmacology , 171(20), 4556–4574. https://doi.org/10.1111/bph.12643
- Ganella, D. E., Lee-Kardashyan, L., Luikinga, S. J., Nguyen, D. L. D., Madsen, H. B., Zbukvic, I. C., Coulthard, R., Lawrence, A. J., & Kim, J. H. (2017). Aripiprazole facilitates extinction of conditioned fear in adolescent rats. Frontiers in Behavioral Neuroscience , 11, 76. https://doi.org/10.3389/fnbeh.2017.00076
- Ganella, D. E., Nguyen, L. D., Lee-Kardashyan, L., Kim, L. E., Paolini, A. G., & Kim, J. H. (2018). Neurocircuitry of fear extinction in adult and juvenile rats. Behavioural Brain Research , 351, 161–167. https://doi.org/10.1016/j.bbr.2018.06.001
- Ganella, D. E., Thangaraju, P., Lawrence, A. J., & Kim, J. H. (2016). Fear extinction in 17 day old rats is dependent on metabotropic glutamate receptor 5 signaling. Behavioural Brain Research , 298, 32–36. https://doi.org/10.1016/j.bbr.2014.12.010
- Gerhard, D. M., & Meyer, H. C. (2021). Extinction trial spacing across days differentially impacts fear regulation in adult and adolescent male mice. Neurobiology of Learning and Memory , 186, 107543. https://doi.org/10.1016/j.nlm.2021.107543
- Gerhard, D. M., Meyer, H. C., & Lee, F. S. (2021). An adolescent sensitive period for threat responding: Impacts of stress and sex. Biological Psychiatry , 89(7), 651–658. https://doi.org/10.1016/j.biopsych.2020.10.003
- Gerhard, D. M., Tse, N., Lee, F. S., & Meyer, H. C. (2023). Developmental age and fatty acid amide hydrolase genetic variation converge to mediate fear regulation in female mice. Developmental Psychobiology , 65(6), 1–12. https://doi.org/10.1002/dev.22409
- Glavonic, E., Mitic, M., Francija, E., Petrovic, Z., & Adzic, M. (2023). Sex-specific role of hippocampal NMDA-Erk-mTOR signaling in fear extinction of adolescent mice. Brain Research Bulletin , 192, 156–167. https://doi.org/10.1016/j.brainresbull.2022.11.011
- Gogolla, N., Caroni, P., Lüthi, A., & Herry, C. (2009). Perineuronal nets protect fear memories from erasure. Science , 325, 1258–1261. https://doi.org/10.1126/science.1174146
- Gruene, T. M., Flick, K., Stefano, A., Shea, S. D., & Shansky, R. M. (2015). Sexually divergent expression of active and passive conditioned fear responses in rats. eLife , 4, e11352. https://doi.org/10.7554/elife.11352
- Gunduz-Cinar, O., Castillo, L. I., Xia, M., van Leer, E., Brockway, E. T., Pollack, G. A., Yasmin, F., Bukalo, O., Limoges, A., Oreizi-Esfahani, S., Kondev, V., Báldi, R., Dong, A., Harvey-White, J., Cinar, R., Kunos, G., Li, Y., Zweifel, L. S., Patel, S., & Holmes, A. (2023). A cortico-amygdala neural substrate for endocannabinoid modulation of fear extinction. Neuron , 111(19), 3053–3067. e10. https://doi.org/10.1016/j.neuron.2023.06.023
- Gupta, R. R., Sen, S., Diepenhorst, L. L., Rudick, C. N., & Maren, S. (2001). Estrogen modulates sexually dimorphic contextual fear conditioning and hippocampal long-term potentiation (LTP) in rats. Brain Research , 888(2), 356–365. https://doi.org/10.1016/S0006-8993(00)03116-4
- Guskjolen, A., Kenney, J. W., de la Parra, J., Yeung, B.-R. A., Josselyn, S. A., & Frankland, P. W. (2018). Recovery of “lost” infant memories in mice. Current Biology , 28(14), 2283–2290. e3. https://doi.org/10.1016/j.cub.2018.05.059
- Hampshire, V., & Davis, J. A. (2005). The role of the veterinary staff in mouse breeding colony management. Lab Animal , 34(4), 45–49. https://doi.org/10.1038/laban0405-45
- Handford, C. E., Tan, S., Lawrence, A. J., & Kim, J. H. (2014). The effect of the mGlu5 negative allosteric modulator MTEP and NMDA receptor partial agonist d-cycloserine on Pavlovian conditioned fear. International Journal of Neuropsychopharmacology , 17(9), 1521–1532. https://doi.org/10.1017/S1461145714000303
- Harmon-Jones, S. K., & Richardson, R. (2021). Maternal care, infant fear memory retention, and the moderating role of variations in separation-induced ultrasonic vocalizations. Developmental Psychobiology , 63(6), e22177. https://doi.org/10.1002/dev.22177
- Hartley, C. A., & Lee, F. S. (2015). Sensitive periods in affective development: Nonlinear maturation of fear learning. Neuropsychopharmacology , 40(1), 50–60. https://doi.org/10.1038/npp.2014.179
- Hefner, K., & Holmes, A. (2007). Ontogeny of fear-, anxiety- and depression-related behavior across adolescence in C57BL/6J mice. Behavioural Brain Research , 176(2), 210–215. https://doi.org/10.1016/j.bbr.2006.10.001
- Hefner, K., Whittle, N., Juhasz, J., Norcross, M., Karlsson, R. M., Saksida, L. M., Bussey, T. J., Singewald, N., & Holmes, A. (2008). Impaired fear extinction learning and cortico-amygdala circuit abnormalities in a common genetic mouse strain. Journal of Neuroscience , 28(32), 8074–8085. https://doi.org/10.1523/JNEUROSCI.4904-07.2008
- Herry, C., Ciocchi, S., Senn, V., Demmou, L., Müller, C., & Lüthi, A. (2008). Switching on and off fear by distinct neuronal circuits. Nature , 454(7204), 600–606. https://doi.org/10.1038/nature07166
- Herry, C., Ferraguti, F., Singewald, N., Letzkus, J. J., Ehrlich, I., & Lüthi, A. (2010). Neuronal circuits of fear extinction. European Journal of Neuroscience , 31(4), 599–612. https://doi.org/10.1111/j.1460-9568.2010.07101.x
- Honeycutt, J. A., Demaestri, C., Peterzell, S., Silveri, M. M., Cai, X., Kulkarni, P., Cunningham, M. G., Ferris, C. F., & Brenhouse, H. C. (2020). Altered corticolimbic connectivity reveals sex-specific adolescent outcomes in a rat model of early life adversity. eLife , 9, e52651. https://doi.org/10.7554/eLife.52651
- Hübner, C., Bosch, D., Gall, A., Lüthi, A., & Ehrlich, I. (2014). Ex vivo dissection of optogenetically activated mPFC and hippocampal inputs to neurons in the basolateral amygdala: Implications for fear and emotional memory. Frontiers in Behavioral Neuroscience , 8, 64. https://doi.org/10.3389/fnbeh.2014.00064
- Ishii, D., Matsuzawa, D., Matsuda, S., Tomizawa-Shinohara, H., Sutoh, C., & Shimizu, E. (2019). Spontaneous recovery of fear differs among early–late adolescent and adult male mice. International Journal of Neuroscience , 129(1), 1–9. https://doi.org/10.1080/00207454.2018.1501049
- Ishikawa, J., Nishimura, R., & Ishikawa, A. (2015). Early-life stress induces anxiety-like behaviors and activity imbalances in the medial prefrontal cortex and amygdala in adult rats. European Journal of Neuroscience , 41(4), 442–453. https://doi.org/10.1111/ejn.12825
- Ito, A., Kikusui, T., Takeuchi, Y., & Mori, Y. (2006). Effects of early weaning on anxiety and autonomic responses to stress in rats. Behavioural Brain Research , 171(1), 87–93. https://doi.org/10.1016/j.bbr.2006.03.023
- Jalali, M., Saldanha, F. Y. L., & Jalali, M. (2016). Basic science methods for clinical researchers. Academic Press.
- Jankord, R., Solomon, M. B., Albertz, J., Flak, J. N., Zhang, R., & Herman, J. P. (2011). Stress vulnerability during adolescent development in rats. Endocrinology , 152(2), 629–638. https://doi.org/10.1210/en.2010-0658
- Jasnow, A. M., Schulkin, J., & Pfaff, D. W. (2006). Estrogen facilitates fear conditioning and increases corticotropin- releasing hormone mRNA expression in the central amygdala in female mice. Hormones and Behavior , 49(2), 197–205. https://doi.org/10.1016/j.yhbeh.2005.06.005
- Jia, M., Travaglia, A., Pollonini, G., Fedele, G., & Alberini, C. M. (2018). Developmental changes in plasticity, synaptic, glia, and connectivity protein levels in rat medial prefrontal cortex. Learning and Memory , 25(10), 436–448. https://doi.org/10.1101/lm.047753.118
- Johnson, F. K., Delpech, J. C., Thompson, G. J., Wei, L., Hao, J., Herman, P., Hyder, F., & Kaffman, A. (2018). Amygdala hyper-connectivity in a mouse model of unpredictable early life stress. Translational Psychiatry , 8(1), 49. https://doi.org/10.1038/s41398-018-0092-z
- Josselyn, S. A., & Frankland, P. W. (2012). Infantile amnesia: A neurogenic hypothesis. Learning and Memory , 19(9), 423–433. https://doi.org/10.1101/lm.021311.110
- Kalemaki, K., Velli, A., Christodoulou, O., Denaxa, M., Karagogeos, D., & Sidiropoulou, K. (2022). The developmental changes in intrinsic and synaptic properties of prefrontal neurons enhance local network activity from the second to the third postnatal weeks in mice. Cerebral Cortex , 32(17), 3633–3650. https://doi.org/10.1093/cercor/bhab438
- Kanari, K., Kikusui, T., Takeuchi, Y., & Mori, Y. (2005). Multidimensional structure of anxiety-related behavior in early-weaned rats. Behavioural Brain Research , 156(1), 45–52. https://doi.org/10.1016/j.bbr.2004.05.008
- Keeley, R. J., Trow, J., & McDonald, R. J. (2015). Strain and sex differences in puberty onset and the effects of THC administration on weight gain and brain volumes. Neuroscience , 305, 328–342. https://doi.org/10.1016/j.neuroscience.2015.07.024
- Kikusui, T., Ichikawa, S., & Mori, Y. (2009). Maternal deprivation by early weaning increases corticosterone and decreases hippocampal BDNF and neurogenesis in mice. Psychoneuroendocrinology , 34(5), 762–772. https://doi.org/10.1016/j.psyneuen.2008.12.009
- Kikusui, T., Nakamura, K., Kakuma, Y., & Mori, Y. (2006). Early weaning augments neuroendocrine stress responses in mice. Behavioural Brain Research , 175(1), 96–103. https://doi.org/10.1016/j.bbr.2006.08.007
- Kikusui, T., Takeuchi, Y., & Mori, Y. (2004). Early weaning induces anxiety and aggression in adult mice. Physiology and Behavior , 81(1), 37–42. https://doi.org/10.1016/j.physbeh.2003.12.016
- Kim, J. H., Hamlin, A. S., & Richardson, R. (2009). Fear extinction across development: The involvement of the medial prefrontal cortex as assessed by temporary inactivation and immunohistochemistry. Journal of Neuroscience , 29(35), 10802–10808. https://doi.org/10.1523/JNEUROSCI.0596-09.2009
- Kim, J. H., Li, S., & Richardson, R. (2011). Immunohistochemical analyses of long-term extinction of conditioned fear in adolescent rats. Cerebral Cortex , 21(3), 530–538. https://doi.org/10.1093/cercor/bhq116
- Kim, J. H., McNally, G. P., & Richardson, R. (2006). Recovery of fear memories in rats: Role of gamma-amino butyric acid (GABA) in infantile amnesia. Behavioral Neuroscience , 120(1), 40–48. https://doi.org/10.1037/0735-7044.120.1.40
- Kim, J. H., & Richardson, R. (2007a). A developmental dissociation in reinstatement of an extinguished fear response in rats. Neurobiology of Learning and Memory , 88(1), 48–57. https://doi.org/10.1016/j.nlm.2007.03.004
- Kim, J. H., & Richardson, R. (2007b). A developmental dissociation of context and GABA effects on extinguished fear in rats. Behavioral Neuroscience , 121(1), 131–139. https://doi.org/10.1037/0735-7044.121.1.131
- Kim, J. H., & Richardson, R. (2008). The effect of temporary amygdala inactivation on extinction and reextinction of fear in the developing rat: Unlearning as a potential mechanism for extinction early in development. Journal of Neuroscience , 28(6), 1282–1290. https://doi.org/10.1523/JNEUROSCI.4736-07.2008
- King, E. C., Pattwell, S. S., Glatt, C. E., & Lee, F. S. (2014). Sensitive periods in fear learning and memory. Stress , 17(1), 13–21. https://doi.org/10.3109/10253890.2013.796355
- Klune, C. B., Jin, B., & Denardo, L. A. (2021). Linking mPFC circuit maturation to the developmental regulation of emotional memory and cognitive flexibility. eLife , 10, e64567. https://doi.org/10.7554/eLife.64567
- Knudsen, E. I. (2004). Sensitive periods in the development of the brain and behavior. Journal of Cognitive Neuroscience , 16(8), 1412–1425. https://doi.org/10.1162/0898929042304796
- Kodama, Y., Kikusui, T., Takeuchi, Y., & Mori, Y. (2008). Effects of early weaning on anxiety and prefrontal cortical and hippocampal myelination in male and female Wistar rats. Developmental Psychobiology , 50(4), 332–342. https://doi.org/10.1002/dev.20289
- Kolb, B., Mychasiuk, R., Muhammad, A., Li, Y., Frost, D. O., & Gibb, R. (2012). Experience and the developing prefrontal cortex. Proceedings of the National Academy of Sciences of the United States of America , 109, 17186–17193. https://doi.org/10.1073/pnas.1121251109
- König, B., & Markl, H. (1987). Maternal care in house mice. Behavioral Ecology and Sociobiology , 20(1), 1–9. https://doi.org/10.1007/bf00292161
- Koppensteiner, P., von Itter, R., Melani, R., Galvin, C., Lee, F. S., & Ninan, I. (2019). Diminished fear extinction in adolescents is associated with an altered somatostatin interneuron–mediated inhibition in the infralimbic cortex. Biological Psychiatry , 86(9), 682–692. https://doi.org/10.1016/j.biopsych.2019.04.035
- Koss, W. A., Belden, C. E., Hristov, A. D., & Juraska, J. M. (2014). Dendritic remodeling in the adolescent medial prefrontal cortex and the basolateral amygdala of male and female rats. Synapse , 68(2), 61–72. https://doi.org/10.1002/syn.21716
- Koss, W. A., Lloyd, M. M., Sadowski, R. N., Wise, L. M., & Juraska, J. M. (2015). Gonadectomy before puberty increases the number of neurons and glia in the medial prefrontal cortex of female, but not male, rats. Developmental Psychobiology , 57(3), 305–312. https://doi.org/10.1002/dev.21290
- Krackow, S. (1993). The effect of weaning weight on offspring fitness in wild house mice (Mus musculus domesticus): A preliminary study. Ethology , 95(1), 76–82. https://doi.org/10.1111/j.1439-0310.1993.tb00458.x
- Krackow, S., & Hoeck, H. N. (1989). Sex ratio manipulation, maternal investment and behaviour during concurrent pregnancy and lactation in house mice. Animal Behaviour , 37, 177–186. https://doi.org/10.1016/0003-3472(89)90108-5
- Kreiker, M., Perez, K., & Brown, K. L. (2021). The effects of early weaning on Pavlovian fear conditioning in young rats. Developmental Psychobiology , 63(6), e22133. https://doi.org/10.1002/dev.22133
- Kroon, T., van Hugte, E., van Linge, L., Mansvelder, H. D., & Meredith, R. M. (2019). Early postnatal development of pyramidal neurons across layers of the mouse medial prefrontal cortex. Scientific Reports , 9(1), 5037. https://doi.org/10.1038/s41598-019-41661-9
- Kucharski, D., & Spear, N. E. (1984). Conditioning of aversion to an odor paired with peripheral shock in the developing rat. Developmental Psychobiology , 17(5), 465–479. https://doi.org/10.1002/dev.420170505
- Kutlu, M. G., Zeid, D., Tumolo, J. M., & Gould, T. J. (2018). Pre-adolescent and adolescent mice are less sensitive to the effects of acute nicotine on extinction and spontaneous recovery. Brain Research Bulletin , 138, 50–55. https://doi.org/10.1016/j.brainresbull.2017.06.010
- Ladd, C., Owens, M. J., & Nemeroff, C. B. (1996). Persistent changes in corticotropin-releasing factor neuronal systems induced by maternal deprivation. Endocrinology , 137(4), 1212–1218. https://doi.org/10.1210/endo.137.4.8625891
- Lanjewar, A. L., Levitt, P., & Eagleson, K. L. (2023). Developmental and molecular contributions to contextual fear memory emergence in mice. BioRxiv , https://doi.org/10.1101/2023.02.03.527024
- Laroche, J., Gasbarro, L., Herman, J. P., & Blaustein, J. D. (2009). Reduced behavioral response to gonadal hormones in mice shipped during the peripubertal/adolescent period. Endocrinology , 150(5), 2351–2358. https://doi.org/10.1210/en.2008-1595
- Latham, N. R., & Mason, G. J. (2008). Maternal deprivation and the development of stereotypic behaviour. Applied Animal Behaviour Science , 110(1–2), 84–108. https://doi.org/10.1016/j.applanim.2007.03.026
- Lawson, K., Scarlata, M. J., Cho, W. C., Mangan, C., Petersen, D., Thompson, H. M., Ehnstrom, S., Mousley, A. L., Bezek, J. L., & Bergstrom, H. C. (2022). Adolescence alcohol exposure impairs fear extinction and alters medial prefrontal cortex plasticity. Neuropharmacology , 211, 109048. https://doi.org/10.1016/j.neuropharm.2022.109048
- Lebron-Milad, K., & Milad, M. R. (2012). Sex differences, gonadal hormones and the fear extinction network: Implications for anxiety disorders. Biology of Mood & Anxiety Disorders, 2(1), 3. https://doi.org/10.1186/2045-5380-2-3
- Ledgerwood, L., Richardson, R., & Cranney, J. (2005). d-Cycloserine facilitates extinction of learned fear: Effects on reacquisition and generalized extinction. Biological Psychiatry , 57(8), 841–847. https://doi.org/10.1016/j.biopsych.2005.01.023
- Ledoux, J. E. (2000). Emotion circuits in the brain. Annual Review of Neuroscience , 23, 155–184. https://doi.org/10.1146/annurev.neuro.23.1.155
- Li, L., Gao, X., & Zhou, Q. (2018). Absence of fear renewal and functional connections between prefrontal cortex and hippocampus in infant mice. Neurobiology of Learning and Memory , 152, 1–9. https://doi.org/10.1016/j.nlm.2018.04.011
- Li, S., Kim, J. H., & Richardson, R. (2012). Differential involvement of the medial prefrontal cortex in the expression of learned fear across development. Behavioral Neuroscience , 126(2), 217–225. https://doi.org/10.1037/a0027151
- Lin, E. J. D., Sun, M., Choi, E. Y., Magee, D., Stets, C. W., & During, M. J. (2015). Social overcrowding as a chronic stress model that increases adiposity in mice. Psychoneuroendocrinology , 51, 318–330. https://doi.org/10.1016/j.psyneuen.2014.10.007
- Liu, W., Ge, T., Leng, Y., Pan, Z., Fan, J., Yang, W., & Cui, R. (2017). The role of neural plasticity in depression: From hippocampus to prefrontal cortex. Neural Plasticity , 2017, 6871089. https://doi.org/10.1155/2017/6871089
- Livia Terranova, M., & Laviola, G. (1995). Individual differences in mouse behavioural development: Effects of precocious weaning and ongomg sexual segregation. Animal Behaviour , 50(5), 1261–1271. https://doi.org/10.1016/0003-3472(95)80042-5
- Luikinga, S. J., Perry, C. J., Madsen, H. B., Lawrence, A. J., & Kim, J. H. (2019). Effects of methamphetamine exposure on fear learning and memory in adult and adolescent rats. Neurochemical Research , 44(9), 2081–2091. https://doi.org/10.1007/s11064-019-02845-x
- MacPherson, K., Whittle, N., Camp, M., Gunduz-Cinar, O., Singewald, N., & Holmes, A. (2013). Temporal factors in the extinction of fear in inbred mouse strains differing in extinction efficacy. Biology of Mood & Anxiety Disorders, 3(1), 13. https://doi.org/10.1186/2045-5380-3-13
- Makowska, I. J., Franks, B., El-Hinn, C., Jorgensen, T., & Weary, D. M. (2019). Standard laboratory housing for mice restricts their ability to segregate space into clean and dirty areas. Scientific Reports , 9(1), 1–10. https://doi.org/10.1038/s41598-019-42512-3
- Malter Cohen, M., Jing, D., Yang, R. R., Tottenham, N., Lee, F. S., & Casey, B. J. (2013). Early-life stress has persistent effects on amygdala function and development in mice and humans. Proceedings of the National Academy of Sciences of the United States of America , 110(45), 18274–18278. https://doi.org/10.1073/pnas.1310163110
- Marek, R., Strobel, C., Bredy, T. W., & Sah, P. (2013). The amygdala and medial prefrontal cortex: Partners in the fear circuit. Journal of Physiology , 591(10), 2381–2391. https://doi.org/10.1113/jphysiol.2012.248575
- Maren, S. (2003). What the amygdala does and doesn't do in aversive learning. Learning and Memory , 10(5), 306–308. https://doi.org/10.1101/lm.68403
- Maren, S., Aharonov, G., & Fanselow, M. S. (1996). Retrograde abolition of conditional fear after excitotoxic lesions in the basolateral amygdala of rats: Absence of a temporal gradient. Behavioral Neuroscience , 110(4), 718–726. https://doi.org/10.1037/0735-7044.110.4.718
- Mathis, A., Mamidanna, P., Cury, K. M., Abe, T., Murthy, V. N., Mathis, M. W., & Bethge, M. (2018). DeepLabCut: Markerless pose estimation of user-defined body parts with deep learning. Nature Neuroscience , 21(9), 1281–1289. https://doi.org/10.1038/s41593-018-0209-y
- Matsuda, S. (2023). Importance of home cage condition for contextual fear memory, fear extinction and spontaneous recovery: Cage size and bedding material. Neuroscience Letters , 804, 137204. https://doi.org/10.1016/j.neulet.2023.137204
- Matus-Amat, P., Higgins, E. A., Barrientos, R. M., & Rudy, J. W. (2004). The role of the dorsal hippocampus in the acquisation and retrieval of context memory representations. Journal of Neuroscience , 24(10), 2431–2439. https://doi.org/10.1523/JNEUROSCI.1598-03.2004
- McCallum, J., Kim, J. H., & Richardson, R. (2010). Impaired extinction retention in adolescent rats: Effects of d-cycloserine. Neuropsychopharmacology , 35(10), 2134–2142. https://doi.org/10.1038/npp.2010.92
- McCarthy, M. M., Nugent, B. M., & Lenz, K. M. (2017). Neuroimmunology and neuroepigenetics in the establishment of sex differences in the brain. Nature Reviews Neuroscience , 18(8), 471–484. https://doi.org/10.1038/nrn.2017.61
- McCormick, C. M., Mongillo, D. L., & Simone, J. J. (2013). Age and adolescent social stress effects on fear extinction in female rats. Stress , 16(6), 678–688. https://doi.org/10.3109/10253890.2013.840283
- McDermott, C. M., Liu, D., & Schrader, L. A. (2012). Role of gonadal hormones in anxiety and fear memory formation and inhibition in male mice. Physiology and Behavior , 105(5), 1168–1174. https://doi.org/10.1016/j.physbeh.2011.12.016
- McEwen, B. S., & Morrison, J. H. (2013). The brain on stress: Vulnerability and plasticity of the prefrontal cortex over the life course. Neuron , 79(1), 16–29. https://doi.org/10.1016/j.neuron.2013.06.028
- McEwen, B. S., Nasca, C., & Gray, J. D. (2016). Stress effects on neuronal structure: Hippocampus, amygdala, and prefrontal cortex. Neuropsychopharmacology , 41(1), 3–23. https://doi.org/10.1038/npp.2015.171
- Mendl, M., & Paul, E. S. (1990). Parental care, sibling relationships and the development of aggressive behaviour in two lines of wild house mice. Behaviour , 116, 11–41. https://doi.org/10.1163/156853990X00347
- Milad, M. R., Igoe, S. A., Lebron-Milad, K., & Novales, J. E. (2009). Estrous cycle phase and gonadal hormones influence conditioned fear extinction. Neuroscience , 164(3), 887–895. https://doi.org/10.1016/j.neuroscience.2009.09.011
- Milad, M. R., & Quirk, G. J. (2002). Neurons in medial prefrontal cortex signal memory for fear extinction. Nature , 420, 70–74. https://doi.org/10.1038/nature01138
- Miller, E. K., & Cohen, J. D. (2001). An integrative theory of prefrontal cortex function. Annual Review of Neuroscience , 24, 167–202. https://doi.org/10.1146/annurev.neuro.24.1.167
- Mitchell, J. R., Trettel, S. G., Li, A. J., Wasielewski, S., Huckleberry, K. A., Fanikos, M., Golden, E., Laine, M. A., & Shansky, R. M. (2022). Darting across space and time: Parametric modulators of sex-biased conditioned fear responses. Learning and Memory , 29(7), 171–180. https://doi.org/10.1101/lm.053587.122
- Moehring, F., O'Hara, C. L., & Stucky, C. L. (2016). Bedding material affects mechanical thresholds, heat thresholds, and texture preference. Journal of Pain , 17(1), 50–64. https://doi.org/10.1016/j.jpain.2015.08.014
- Mogi, K., Ishida, Y., Nagasawa, M., & Kikusui, T. (2016). Early weaning impairs fear extinction and decreases brain-derived neurotrophic factor expression in the prefrontal cortex of adult male C57BL/6 mice. Developmental Psychobiology , 58(8), 1034–1042. https://doi.org/10.1002/dev.21437
- Morgan, M. A., & Pfaff, D. W. (2001). Effects of estrogen on activity and fear-related behaviors in mice. Hormones and Behavior , 40(4), 472–482. https://doi.org/10.1006/hbeh.2001.1716
- Moriceau, S., Shionoya, K., Jakubs, K., & Sullivan, R. M. (2009). Early-life stress disrupts attachment learning: The role of amygdala corticosterone, locus ceruleus corticotropin releasing hormone, and olfactory bulb norepinephrine. Journal of Neuroscience , 29(50), 15745–15755. https://doi.org/10.1523/JNEUROSCI.4106-09.2009
- Moriceau, S., & Sullivan, R. M. (2004). Corticosterone influences on mammalian neonatal sensitive-period learning. Behavioral Neuroscience , 118(2), 274–281. https://doi.org/10.1037/0735-7044.118.2.274
- Moriceau, S., & Sullivan, R. M. (2006). Maternal presence serves as a switch between learning fear and attraction in infancy. Nature Neuroscience , 9(8), 1004–1006. https://doi.org/10.1038/nn1733
- Mukherjee, A., & Caroni, P. (2018). Infralimbic cortex is required for learning alternatives to prelimbic promoted associations through reciprocal connectivity. Nature Communications , 9(1), 2727. https://doi.org/10.1038/s41467-018-05318-x
- Muller, J., Corodimas, K. P., Fridel, Z., & LeDoux, J. E. (1997). Functional inactivation of the lateral and basal nuclei of the amygdala by muscimol infusion prevents fear conditioning to an explicit conditioned stimulus and to contextual stimuli. Behavioral Neuroscience , 111(4), 683–691. https://doi.org/10.1037/0735-7044.111.4.683
- Myers, K. M., & Davis, M. (2007). Mechanisms of fear extinction. Molecular Psychiatry , 12(2), 120–150. https://doi.org/10.1038/sj.mp.4001939
- Nakamura, K., Kikusui, T., Takeuchi, Y., & Mori, Y. (2003). The influence of early weaning on aggressive behavior in mice. Journal of Veterinary Medical Science , 65(12), 1347–1349. https://doi.org/10.1292/jvms.65.1347
- Nelson, J. F., Karelus, K., Felicio, L. S., & Johnson, T. E. (1990). Genetic influences on the timing of puberty in mice. Biology of Reproduction , 42(4), 649–655. https://doi.org/10.1095/biolreprod42.4.649
- Nieves, G. M., Bravo, M., Baskoylu, S., & Bath, K. G. (2020). Early life adversity decreases pre-adolescent fear expression by accelerating amygdala PV cell development. eLife , 9, 1–24. https://doi.org/10.7554/eLife.55263
- Ono, M., Kikusui, T., Sasaki, N., Ichikawa, M., Mori, Y., & Murakami-Murofushi, K. (2008). Early weaning induces anxiety and precocious myelination in the anterior part of the basolateral amygdala of male Balb/c mice. Neuroscience , 156(4), 1103–1110. https://doi.org/10.1016/j.neuroscience.2008.07.078
- Oomen, C. A., Soeters, H., Audureau, N., Vermunt, L., van Hasselt, F. N., Manders, E. M. M., Joëls, M., Lucassen, P. J., & Krugers, H. (2010). Severe early life stress hampers spatial learning and neurogenesis, but improves hippocampal synaptic plasticity and emotional learning under high-stress conditions in adulthood. Journal of Neuroscience , 30(19), 6635–6645. https://doi.org/10.1523/JNEUROSCI.0247-10.2010
- Orsini, C. A., & Maren, S. (2012). Neural and cellular mechanisms of fear and extinction memory formation. Neuroscience and Biobehavioral Reviews , 36(7), 1773–1802. https://doi.org/10.1016/j.neubiorev.2011.12.014
- Oštádalová, I., & Babický, A. (2012). Periodization of the early postnatal development in the rat with particular attention to the weaning period. Physiological Research , 61, S1–S7. https://doi.org/10.33549/physiolres.932385
- Owen, E. H., Logue, S. F., Rasmussen, D. L., & Wehner, J. M. (1997). Assessment of learning by the Morris water task and fear conditioning in inbred mouse strains and F1 hybrids: Implications of genetic background for single gene mutations and quantitative trait loci analyses. Neuroscience , 80(4), 1087–1099. https://doi.org/10.1016/S0306-4522(97)00165-6
- Pape, H. C., & Pare, D. (2010). Plastic synaptic networks of the amygdala for the acquisition, expression, and extinction of conditioned fear. Physiological Reviews , 90(2), 419–463. https://doi.org/10.1152/physrev.00037.2009
- Park, C. H. J., Ganella, D. E., & Kim, J. H. (2017a). A dissociation between renewal and contextual fear conditioning in juvenile rats. Developmental Psychobiology , 59(4), 515–522. https://doi.org/10.1002/dev.21516
- Park, C. H. J., Ganella, D. E., & Kim, J. H. (2017b). Juvenile female rats, but not male rats, show renewal, reinstatement, and spontaneous recovery following extinction of conditioned fear. Learning and Memory , 24(12), 630–636. https://doi.org/10.1101/lm.045831.117
- Park, C. H. J., Ganella, D. E., & Kim, J. H. (2020). Context fear learning and renewal of extinguished fear are dissociated in juvenile female rats. Developmental Psychobiology , 62(1), 123–129. https://doi.org/10.1002/dev.21888
- Park, C. H. J., Ganella, D. E., Perry, C. J., & Kim, J. H. (2020). Dissociated roles of dorsal and ventral hippocampus in recall and extinction of conditioned fear in male and female juvenile rats. Experimental Neurology , 329, 113306. https://doi.org/10.1016/j.expneurol.2020.113306
- Pattwell, S. S., Duhoux, S., Hartley, C. A., Johnson, D. C., Jing, D., Elliott, M. D., Ruberry, E. J., Powers, A., Mehta, N., Yang, R. R., Soliman, F., Glatt, C. E., Casey, B. J., Ninan, I., & Lee, F. S. (2012). Altered fear learning across development in both mouse and human. Proceedings of the National Academy of Sciences of the United States of America , 109(40), 16318–16323. https://doi.org/10.1073/pnas.1206834109
- Pattwell, S. S., Liston, C., Jing, D., Ninan, I., Yang, R. R., Witztum, J., Murdock, M. H., Dincheva, I., Bath, K. G., Casey, B. J., Deisseroth, K., & Lee, F. S. (2016). Dynamic changes in neural circuitry during adolescence are associated with persistent attenuation of fear memories. Nature Communications , 7, 11475. https://doi.org/10.1038/ncomms11475
- Paylor, R., Tracy, R., Wehner, J., & Rudy, J. W. (1994). DBA/2 and C57BL/6 mice differ in contextual fear but not auditory fear conditioning. Behavioral Neuroscience , 108(4), 810–817. https://doi.org/10.1037/0735-7044.108.4.810
- Perry, C., Ganella, D., Nguyen, L., Du, X., Drummond, K., Whittle, S., Pang, T., & Kim, J. H. (2020). Assessment of conditioned fear extinction in male and female adolescent rats. Psychoneuroendocrinology , 116, 104670. https://doi.org/10.1016/j.psyneuen.2020.104670
- Phelps, E. A., & LeDoux, J. E. (2005). Contributions of the amygdala to emotion processing: From animal models to human behavior. Neuron , 48(2), 175–187. https://doi.org/10.1016/j.neuron.2005.09.025
- Phillips, R., & LeDoux, J. (1992). Differential contribution of amygdala and hippocampus to cued and contextual fear conditioning. Behavioral Neuroscience , 106(2), 274–285. https://doi.org/10.1037/0735-7044.106.2.274
- Pibiri, F., Nelson, M., Guidotti, A., Costa, E., & Pinna, G. (2008). Decreased corticolimbic allopregnanolone expression during social isolation enhances contextual fear: A model relevant for posttraumatic stress disorder. Proceedings of the National Academy of Sciences of the United States of America , 105(14), 5567–5572. https://doi.org/10.1073/pnas.0801853105
- Piekarski, D. J., Boivin, J. R., & Wilbrecht, L. (2017). Ovarian hormones organize the maturation of inhibitory neurotransmission in the frontal cortex at puberty onset in female mice. Current Biology , 27(12), 1735–1745. e3. https://doi.org/10.1016/j.cub.2017.05.027
- Premachandran, H., Zhao, M., & Arruda-Carvalho, M. (2020). Sex differences in the development of the rodent corticolimbic system. Frontiers in Neuroscience , 14, 583477. https://doi.org/10.3389/fnins.2020.583477
- Quiñones, M. M., Maldonado, L., Velazquez, B., & Porter, J. T. (2016). Candesartan ameliorates impaired fear extinction induced by innate immune activation. Brain, Behavior, and Immunity , 52, 169–177. https://doi.org/10.1016/j.bbi.2015.10.017
- Quirk, G. J., & Mueller, D. (2008). Neural mechanisms of extinction learning and retrieval. Neuropsychopharmacology , 33(1), 56–72. https://doi.org/10.1038/sj.npp.1301555
- Radulovic, J., Kammermeier, J., & Spiess, J. (1998). Generalization of fear responses in C57BL/6N mice subjected to one-trial foreground contextual fear conditioning. Behavioural Brain Research , 95(2), 179–189. https://doi.org/10.1016/S0166-4328(98)00039-4
- Raineki, C., Holman, P. J., Debiec, J., Bugg, M., Beasley, A., & Sullivan, R. M. (2010). Functional emergence of the hippocampus in context fear learning in infant rats. Hippocampus , 20(9), 1037–1046. https://doi.org/10.1002/hipo.20702
- Ramsaran, A. I., Schlichting, M. L., & Frankland, P. W. (2018). The ontogeny of memory persistence and specificity. Developmental Cognitive Neuroscience , 36(2019), 100591. https://doi.org/10.1016/j.dcn.2018.09.002
- Ramsaran, A. I., Wang, Y., Golbabaei, A., Aleshin, S., de Snoo, M. L., Yeung, B. R. A., Rashid, A. J., Awasthi, A., Lau, J., Tran, L. M., Ko, S. Y., Abegg, A., Duan, L. C., McKenzie, C., Gallucci, J., Ahmed, M., Kaushik, R., Dityatev, A., Josselyn, S. A., & Frankland, P. W. (2023). A shift in the mechanisms controlling hippocampal engram formation during brain maturation. Science , 380(6644), 543–551. https://doi.org/10.1126/science.ade6530
- Reiss, D., Wolter-Sutter, A., Krezel, W., & Ouagazzal, A. M. (2007). Effects of social crowding on emotionality and expression of hippocampal nociceptin/orphanin FQ system transcripts in mice. Behavioural Brain Research , 184(2), 167–173. https://doi.org/10.1016/j.bbr.2007.07.010
- Rescorla, R. A., & Heth, C. D. (1975). Reinstatement of fear to an extinguished conditioned stimulus. Journal of Experimental Psychology: Animal Behavior Processes , 1(1), 88–96. https://doi.org/10.1037/0097-7403.1.1.88
- Revillo, D. A., Castello, S., Paglini, G., & Arias, C. (2014). Reacquisition, reinstatement, and renewal of a conditioned taste aversion in preweanling rats. Developmental Psychobiology , 56(4), 713–725. https://doi.org/10.1002/dev.21140
- Revillo, D. A., Cotella, E., Paglini, M. G., & Arias, C. (2015). Contextual learning and context effects during infancy: 30years of controversial research revisited. Physiology and Behavior , 148, 6–21. https://doi.org/10.1016/j.physbeh.2015.02.012
- Revillo, D. A., Paglini, M. G., & Arias, C. (2014). Spontaneous recovery from extinction in the infant rat. Behavioural Brain Research , 274, 149–157. https://doi.org/10.1016/j.bbr.2014.08.009
- Revillo, D. A., Trebucq, G., Paglini, M. G., & Arias, C. (2016). Reinstatement of an extinguished fear conditioned response in infant rats. Learning and Memory , 23(1), 1–8. https://doi.org/10.1101/lm.038919.115
- Richter, S. H., Kästner, N., Loddenkemper, D. H., Kaiser, S., & Sachser, N. (2016). A time to wean? Impact of weaning age on anxiety-like behaviour and stability of behavioural traits in full adulthood. PLoS ONE , 11(12), 1–21. https://doi.org/10.1371/journal.pone.0167652
- Riddle, M. C., McKenna, M. C., Yoon, Y. J., Pattwell, S. S., Santos, P. M. G., Casey, B. J., & Glatt, C. E. (2013). Caloric restriction enhances fear extinction learning in mice. Neuropsychopharmacology , 38(6), 930–937. https://doi.org/10.1038/npp.2012.268
- Robinson, I., Dowdall, T., & Meert, T. F. (2004). Development of neuropathic pain is affected by bedding texture in two models of peripheral nerve injury in rats. Neuroscience Letters , 368(1), 107–111. https://doi.org/10.1016/j.neulet.2004.06.078
- Romeo, R. D. (2010). Pubertal maturation and programming of hypothalamic-pituitary-adrenal reactivity. Frontiers in Neuroendocrinology , 31(2), 232–240. https://doi.org/10.1016/j.yfrne.2010.02.004
- Romeo, R. D., & McEwen, B. S. (2006). Stress and the adolescent brain. Annals of the New York Academy of Sciences , 1094(1), 202–214. https://doi.org/10.1196/annals.1376.022
- Roozendaal, B., McEwen, B. S., & Chattarji, S. (2009). Stress, memory and the amygdala. Nature Reviews Neuroscience , 10(6), 423–433. https://doi.org/10.1038/nrn2651
- Rozeske, R. R., Valerio, S., Chaudun, F., & Herry, C. (2015). Prefrontal neuronal circuits of contextual fear conditioning. Genes, Brain and Behavior , 14(1), 22–36. https://doi.org/10.1111/gbb.12181
- Rubinow, M. J., & Juraska, J. M. (2009). Neuron and glia numbers in the basolateral nucleus of the amygdala from preweaning through old age in male and female rats: A stereological study. Journal of Comparative Neurology , 512(6), 717–725. https://doi.org/10.1002/cne.21924
- Rudy, J. W., Barrientos, R. M., & O'Reilly, R. C. (2002). Hippocampal formation supports conditioning to memory of a context. Behavioral Neuroscience , 116(4), 530–538. https://doi.org/10.1037/0735-7044.116.4.530
- Rudy, J. W., & Morledge, P. (1994). Ontogeny of contextual fear conditioning in rats: Implications for consolidation, infantile amnesia, and hippocampal system function. Behavioral Neuroscience , 108(2), 227–234. https://doi.org/10.1037/0735-7044.108.2.227
- Sachser, N., Zimmermann, T. D., Hennessy, M. B., & Kaiser, S. (2020). Sensitive phases in the development of rodent social behavior. Current Opinion in Behavioral Sciences , 36, 63–70. https://doi.org/10.1016/j.cobeha.2020.07.014
- Samifanni, R., Zhao, M., Cruz-Sanchez, A., Satheesh, A., Mumtaz, U., & Arruda-Carvalho, M. (2021). Developmental emergence of persistent memory for contextual and auditory fear in mice. Learning and Memory , 28(11), 414–421. https://doi.org/10.1101/LM.053471.121
- Sanders, M. J., Wiltgen, B. J., & Fanselow, M. S. (2003). The place of the hippocampus in fear conditioning. European Journal of Pharmacology , 463(1–3), 217–223. https://doi.org/10.1016/S0014-2999(03)01283-4
- Schiffino, F. L., Murawski, N. J., Rosen, J. B., & Stanton, M. E. (2011). Ontogeny and neural substrates of the context preexposure facilitation effect. Neurobiology of Learning and Memory , 95(2), 190–198. https://doi.org/10.1016/j.nlm.2010.11.011
- Schneider, M. (2013). Adolescence as a vulnerable period to alter rodent behavior. Cell and Tissue Research , 354(1), 99–106. https://doi.org/10.1007/s00441-013-1581-2
- Seemiller, L. R., Mooney-Leber, S. M., Henry, E., McGarvey, A., Druffner, A., Peltz, G., & Gould, T. J. (2021). Genetic background determines behavioral responses during fear conditioning. Neurobiology of Learning and Memory , 184, 107501. https://doi.org/10.1016/j.nlm.2021.107501
- Selleck, R. A., Zhang, W., Samberg, H. D., Padival, M., & Rosenkranz, J. A. (2018). Limited prefrontal cortical regulation over the basolateral amygdala in adolescent rats. Scientific Reports , 8(1), 1–16. https://doi.org/10.1038/s41598-018-35649-0
- Shansky, R. M. (2018). Sex differences in behavioral strategies: Avoiding interpretational pitfalls. Current Opinion in Neurobiology , 49, 95–98. https://doi.org/10.1016/j.conb.2018.01.007
- Shimozuru, M., Kodama, Y., Iwasa, T., Kikusui, T., Takeuchi, Y., & Mori, Y. (2007). Early weaning decreases play-fighting behavior during the postweaning developmental period of Wistar rats. Developmental Psychobiology , 49(4), 343–350. https://doi.org/10.1002/dev.20202
- Shin, S., & Lee, S. (2023). The impact of environmental factors during maternal separation on the behaviors of adolescent C57BL/6 mice. Frontiers in Molecular Neuroscience , 16, 1147951. https://doi.org/10.3389/fnmol.2023.1147951
- Siegmund, A., Langnaese, K., & Wotjak, C. T. (2005). Differences in extinction of conditioned fear in C57BL/6 substrains are unrelated to expression of α-synuclein. Behavioural Brain Research , 157(2), 291–298. https://doi.org/10.1016/j.bbr.2004.07.007
- Siegmund, A., & Wotjak, C. T. (2007). A mouse model of posttraumatic stress disorder that distinguishes between conditioned and sensitised fear. Journal of Psychiatric Research , 41(10), 848–860. https://doi.org/10.1016/j.jpsychires.2006.07.017
- Sierra-Mercado, D., Corcoran, K. A., Lebrón-Milad, K., & Quirk, G. J. (2006). Inactivation of the ventromedial prefrontal cortex reduces expression of conditioned fear and impairs subsequent recall of extinction. European Journal of Neuroscience , 24(6), 1751–1758. https://doi.org/10.1111/j.1460-9568.2006.05014.x
- Sotres-Bayon, F., & Quirk, G. J. (2010). Prefrontal control of fear: More than just extinction. Current Opinion in Neurobiology , 20(2), 231–235. https://doi.org/10.1016/j.conb.2010.02.005.Prefrontal
- Spear, L. P. (2000). The adolescent brain and age-related behavioral manifestations. Neuroscience and Biobehavioral Reviews , 24(4), 417–463. https://doi.org/10.1016/S0149-7634(00)00014-2
- Stafford, J. M., Maughan, D. A. K., Ilioi, E. C., & Lattal, K. M. (2013). Exposure to a fearful context during periods of memory plasticity impairs extinction via hyperactivation of frontal-amygdalar circuits. Learning and Memory , 20(3), 156–163. https://doi.org/10.1101/lm.029801.112
- Stiedl, O., Radulovic, J., Lohmann, R., Birkenfeld, K., Palve, M., Kammermeier, J., Sananbenesi, F., & Spiess, J. (1999). Strain and substrain differences in context- and tone-dependent fear conditioning of inbred mice. Behavioural Brain Research , 104(1–2), 1–12. https://doi.org/10.1016/S0166-4328(99)00047-9
- Stylianakis, A. A., Baker, K. D., & Richardson, R. (2022). Pharmacological enhancement of extinction retention in non-stressed adolescent rats but not those exposed to chronic corticosterone. Frontiers in Neuroscience , 16, 822709. https://doi.org/10.3389/fnins.2022.822709
- Stylianakis, A. A., Richardson, R., & Baker, K. D. (2019). Timing is everything: Developmental differences in the effect of chronic corticosterone exposure on extinction retention. Behavioral Neuroscience , 133(5), 467–477. https://doi.org/10.1037/bne0000326
- Sullivan, R. M., Landers, M., Yeaman, B., & Wilson, D. A. (2000). Neurophysiology: Good memories of bad events in infancy. Nature , 407(6800), 38. https://doi.org/10.1038/35024156
- Sullivan, R. M., & Wilson, D. A. (1994). The locus coeruleus, norepinephrine, and memory in newborns. Brain Research Bulletin , 35(5–6), 467–472. https://doi.org/10.1016/0361-9230(94)90160-0
- Swann, J. W., Brady, R. J., & Martin, D. L. (1989). Postnatal development of GABA-mediated synaptic inhibition in rat hippocampus. Neuroscience , 28(3), 551–561. https://doi.org/10.1016/0306-4522(89)90004-3
- Takahashi, L. K. (1994). Organizing action of corticosterone on the development of behavioral inhibition in the preweanling rat. Developmental Brain Research , 81(1), 121–127. https://doi.org/10.1016/0165-3806(94)90074-4
- Takita, M., & Kikusui, T. (2016). Early weaning influences short-term synaptic plasticity in the medial prefrontal-anterior basolateral amygdala pathway. Neuroscience Research , 103, 48–53. https://doi.org/10.1016/j.neures.2015.08.003
- Tallot, L., Doyère, V., & Sullivan, R. M. (2016). Developmental emergence of fear/threat learning: Neurobiology, associations and timing. Genes, Brain and Behavior , 15(1), 144–154. https://doi.org/10.1111/gbb.12261
- Terranova, M. L., & Laviola, G. (2001). ∂-Opioid modulation of social interactions in juvenile mice weaned at different ages. Physiology and Behavior , 73(3), 393–400. https://doi.org/10.1016/S0031-9384(01)00447-4
- Travaglia, A., Bisaz, R., Cruz, E., & Alberini, C. M. (2016). Developmental changes in plasticity, synaptic, glia and connectivity protein levels in rat dorsal hippocampus. Neurobiology of Learning and Memory , 135, 125–138. https://doi.org/10.1016/j.nlm.2016.08.005
- Travaglia, A., Bisaz, R., Sweet, E. S., Blitzer, R. D., & Alberini, C. M. (2016). Infantile amnesia reflects a developmental critical period for hippocampal learning. Nature Neuroscience , 19(9), 1225–1233. https://doi.org/10.1038/nn.4348
- Trivers, R. L., & Willard, D. E. (1973). Natural selection of parental ability to vary the sex ratio of offspring published by: American association for the advancement of science stable. Natural selection of parental ability to vary the sex ratio of off. Science , 179(4068), 90–92. https://doi.org/10.1126/science.179.4068.90
- van Eden, C. G., & Uylings, H. B. M. (1985). Cytoarchitectonic development of the prefrontal cortex in the rat. Journal of Comparative Neurology , 241(3), 253–267. https://doi.org/10.1002/cne.902410302
- Vetter-O'Hagen, C. S., & Spear, L. P. (2012). Hormonal and physical markers of puberty and their relationship to adolescent-typical novelty-directed behavior. Developmental Psychobiology , 54(5), 523–535. https://doi.org/10.1002/dev.20610
- Vidal-Gonzalez, I., Vidal-Gonzalez, B., Rauch, S. L., & Quirk, G. J. (2006). Microstimulation reveals opposing influences of prelimbic and infralimbic cortex on the expression of conditioned fear. Learning and Memory , 13(6), 728–733. https://doi.org/10.1101/lm.306106
- Walker, D. M., Bell, M. R., Flores, C., Gulley, J. M., Willing, J., & Paul, M. J. (2017). Adolescence and reward: Making sense of neural and behavioral changes amid the chaos. Journal of Neuroscience , 37(45), 10855–10866. https://doi.org/10.1523/JNEUROSCI.1834-17.2017
- Würbel, H., & Stauffacher, M. (1997). Age and weight at weaning affect corticosterone level and development of stereotypies in ICR-mice. Animal Behaviour , 53(5), 891–900. https://doi.org/10.1006/anbe.1996.0424
- Xu, C., Krabbe, S., Gründemann, J., Botta, P., Fadok, J. P., Osakada, F., Saur, D., Grewe, B. F., Schnitzer, M. J., Callaway, E. M., & Lüthi, A. (2016). Distinct hippocampal pathways mediate dissociable roles of context in memory retrieval. Cell , 167(4), 961–972. e16. https://doi.org/10.1016/j.cell.2016.09.051
- Yap, C. S. L., & Richardson, R. (2007). Extinction in the developing rat: An examination of renewal effects. Developmental Psychobiology , 49(6), 565–575. https://doi.org/10.1002/dev.20244
- Yohn, N. L., & Blendy, J. A. (2017). Adolescent chronic unpredictable stress exposure is a sensitive window for long-term changes in adult behavior in mice. Neuropsychopharmacology , 42(8), 1670–1678. https://doi.org/10.1038/npp.2017.11
- Zbukvic, I. C., Park, C. H. J., Ganella, D. E., Lawrence, A. J., & Kim, J. H. (2017). Prefrontal dopaminergic mechanisms of extinction in adolescence compared to adulthood in rats. Frontiers in Behavioral Neuroscience , 11, 32. https://doi.org/10.3389/fnbeh.2017.00032
- Zhang, W., & Rosenkranz, J. A. (2013). Repeated restraint stress enhances cue-elicited conditioned freezing and impairs acquisition of extinction in an age-dependent manner. Behavioural Brain Research , 248, 12–24. https://doi.org/10.1016/j.bbr.2013.03.028
- Zimmermann, K. S., Richardson, R., & Baker, K. D. (2019). Maturational changes in prefrontal and amygdala circuits in adolescence: Implications for understanding fear inhibition during a vulnerable period of development. Brain Sciences , 9(3), 65. https://doi.org/10.3390/brainsci9030065
- Zimmermann, K. S., Richardson, R., & Baker, K. D. (2023). Developmental changes in functional connectivity between the prefrontal cortex and amygdala following fear extinction. Neurobiology of Learning and Memory , 205, 107847. https://doi.org/10.1016/j.nlm.2023.107847