An Experimental Liver Metastasis Mouse Model Suitable for Short and Long-Term Intravital Imaging
Dieuwke L. Marvin, Dieuwke L. Marvin, Peter ten Dijke, Peter ten Dijke, Laila Ritsma, Laila Ritsma
Abstract
The liver is a frequent site of cancer metastasis, but current treatment options for cancer patients with liver metastasis are limited, resulting in poor prognosis. Colonization of the liver by cancer cells is a multistep and temporally controlled process. Investigating this process in biological relevant settings in a dynamic manner may lead to new therapeutic avenues. Experimental mouse models of liver metastasis combined with high-resolution microscopy methods can facilitate study of the mechanisms that underlie the outgrowth of cancer cells in the liver. Intravital imaging can provide information on the behavior of tumor cells in their biological setting, in time frames of hours to days. In this unit, we describe the experimental induction of liver metastasis through administration of cancer cells into mice via mesenteric vein injection. The behavior of these injected cells can then be studied using intravital imaging by surgical exposure or through an abdominal imaging window. The approach is described for use with an upright multiphoton microscope, making it widely applicable. © 2021 The Authors. Current Protocols published by Wiley Periodicals LLC.
Basic Protocol 1 : Inducing liver metastasis through mesenteric vein injection
Basic Protocol 2 : Short-term imaging of tumor cells in mouse liver
Basic Protocol 3 : Long-term imaging of tumor cells in mouse liver using an abdominal imaging window
INTRODUCTION
The presence of metastases dramatically decreases the survival of cancer patients. Thus, to enable better treatment of cancer, it is essential to understand in greater detail the underlying mechanisms controlling the colonization of disseminated cancer cells (Steeg 2016; Welch & Hurst, 2019). The highly vascularized nature of the liver as well as the suitable microenvironment make it a favorable organ for metastatic outgrowth of primary tumor cells (Vidal-Vanaclocha, 2011). Indeed, the liver is a common site of metastasis in many cancer types, including melanoma and colorectal, pancreatic, lung, and breast cancers (Hess et al., 2006), and liver metastases result in a poor prognosis (Landreau et al., 2015). Treatment options for patients presenting with liver metastases in the clinic are limited and mainly consists of surgical resection in the case of single-lobe metastasis. This demonstrates the unmet clinical need: a better understanding of the molecular and cellular determinants of liver metastasis would provide novel avenues for therapy. Mechanistically, metastasis can be subdivided into several steps, including tumor cell migration, dissemination via the vasculature, and colonization into secondary organs (Marvin, Heijboer, ten Dijke, & Ritsma, 2020). Colonization is thought to be a bottleneck of metastasis, as many tumor cells disseminate but only a very few form metastases (Luzzi et al., 1998). As such, obtaining insight into this last step of metastatic colonization is important.
Different mouse models can be used to study liver metastases. Several spontaneous mouse models develop metastases in the liver (Gomez-Cuadrado, Tracey, Ma, Qian, & Brunton, 2017). However, the frequency, location, and timing of where and when these metastases will form can be unpredictable and heterogenous. In contrast, experimental liver metastasis models in which tumor cells are injected directly into the bloodstream lead to more robust and controllable liver metastasis formation.
The portal vein is one of the main blood supply routes for the liver, which can be exploited to induce tumor cell colonization, but is relatively large, and injection into this vein can lead to potentially large volumes of blood loss. To avoid this, mesenteric veins can be used for administration instead (Gül et al., 2014; Van Der Bij et al., 2010). After tumor cells are injected into the mesenteric vein, the liver capillaries are the first capillary network they encounter. After extravasation, the injected tumor cells can colonize the liver. Using this model, metastasis formation in the liver can be studied in a more robust, controlled manner, such as is required to study the effects of genetic determinants in cancer cells, tumor microenvironmental cells, or pharmacological interventions.
For the proper colonization of the liver, adaptation of the tumor cells to the foreign environment is key. To unravel metastatic processes, it is necessary to understand the dynamic processes of both early (extravasation, niche finding) and late steps (micro- and macro-metastasis formation) of liver metastasis formation on a high-resolution and single-cell level. High-resolution intravital microscopy can provide this (Beerling, Ritsma, Vrisekoop, Derksen, & Van Rheenen, 2011), as it enables the tracing of individual, fluorescently labeled cancer cells forming liver metastases over time frames of hours to weeks in living mice (Ritsma et al., 2012; 2013).
In this article, we present methods for inducing liver metastasis through a mesenteric vein injection (Basic Protocol 1) and for studying them at high resolution over time, either over short time frames through surgical exposure (<8 h, Basic Protocol 2) or over long time frames through implantation of an abdominal imaging window (<2 weeks, Basic Protocol 3).
STRATEGIC PLANNING
In setting up this technique, we used the highly metastatic murine B16F10 melanoma cell line (Overwijk & Restifo, 2000; Poste, Doll, Hart, & Fidler, 1980). Other murine cancer cell lines can also be used, albeit in their syngeneic mouse strain to prevent immune rejection of the tumor cells. The speed of liver metastasis formation will be highly dependent on the cell line used. It is thus advisable to perform exploratory experiments to assess the speed at which metastases form in the liver in your system. Depending on the timeline of the experiment and the metastatic potential of the cell line, different amounts of cells can be used. For short-term experiments (<5 days), 0.5 × 106-1 × 106 can be used. For long-term experiments, fewer cells can be used.
Some variation in metastasis formation between mice is to be expected; the approximate variation we have observed is a standard deviation of 1.25 with normal distribution. The number of animals needed should be calculated depending on the expected results, as determined based on previous in vitro or in vivo experiments. We recommend not using more than 10 mice per group due to time considerations for the surgery. Consider the following example, using an unpaired t -test: When expecting a true difference of 2 between one experimental and one control group with a power of 0.8 and a type 1 error probability of 0.05, seven animals will be needed per group in order to be able to reject the null hypothesis that the difference between the two groups is 0. We suggest including one additional mouse per seven mice in an experimental group in case technical difficulties arise with the surgeries or imaging procedure.
To visualize the injected tumor cells, cells should be fluorescently labelled. A very effective method is to transduce the cells before injection with a lentiviral expression plasmid containing either a nuclear or a cytoplasmic fluorophore. We recommend avoiding the human cytomegalovirus (CMV) promoter for the expression of a fluorophore, as this promoter can occasionally be silenced in vivo. Good alternatives are the mouse phosphoglycerate kinase (PGK) or human elongation factor (EF) 1-α promoter. Using tumor cells expressing a nuclear histone 2B (H2B)-attached fluorophore, cell migration, cellular division, or apoptotic events can be observed. Using cytoplasmic fluorophores, shapes of cells and subtle movements can be traced. However, separation of individual cells can easily be lost when cells are densely packed or when micro-metastases grow bigger.
Basic Protocol 1: INDUCING LIVER METASTASIS THROUGH MESENTERIC VEIN INJECTION
This protocol describes the induction of an experimental liver metastasis model through mesenteric vein injection. Before starting these mouse experiments, ethical approval of the local animal ethics committee should be obtained.
Eight-week-old mice are preferred over older mice for this injection due to the smaller size of the mesenteric vein and decreased occurrence of bleeding. However, when an abdominal imaging window is to be implanted (Basic Protocol 3) after the mesenteric vein injection, 10-week-old mice are favored due to their larger size of the liver and higher weight; in addition, female mice are preferred because they are less aggressive, reducing the changes of damage to the window. The cells used in this example were B16F10 (ATCC®CRL-6475™) transduced with a lentiviral plasmid containing a fluorophore attached to H2B.
Materials
-
Murine cancer cells (e.g., B16F10 cells) transduced with lentiviral plasmid expressing a nuclear or cytoplasmic fluorophore, mycoplasma free
-
10% dimethyl sulfoxide (DMSO)/50% fetal bovine serum (FBS)/40% Dulbecco's modified eagle medium (DMEM)
-
Culture medium: DMEM supplemented with 10% fetal bovine serum (FBS) and 100 IU/ml penicillin-streptomycin (cat no. 15140122, Thermo Fisher Scientific)
-
Phosphate-buffered saline (PBS; sterile)
-
Trypsin/EDTA: 5% trypsin/ethylenediaminetetraacetic acid (EDTA; cat no. 15400054, Thermo Fisher Scientific)
-
0.03 mg/ml buprenorphine
-
70% ethanol (EtOH) for sterilization
-
8- to 10-week-old C57BL/6J mice
-
100% (v/v) isoflurane
-
Ophthalmic ointment (Oculentum Simplex 5 g, sterile; cat. no. 220201, Added Pharma)
-
37°C water bath
-
15-ml Falcon tubes (sterile)
-
100/20-mm culture dishes (sterile; cat. no. 664160, Greiner Bio-one)
-
Cell culture hood
-
CO2 incubator
-
Benchtop centrifuge (Hettich® ROTINA 38)
-
Dual-chamber cell counting slides (Bio-Rad)
-
TC20™ Automated Cell Counter (Bio-Rad)
-
1.5-ml microcentrifuge tubes (sterile)
-
Pipetboy Acu 2 (cat. no. 15500, Integra) or equivalent
-
1000- and 10-μl pipets (cat. nos. 17014382 and 17014388, Mettler Toledo)
-
1000-μl pipet tips, sterile
-
1-20 μl pipet tips, sterile
-
Surgical tools:
- Extra-fine Bonn scissors (cat. no. 14084-08, Fine Science Tools)
- Fine scissors (cat. no. 91460-11, Fine Science Tools)
- Halsey needle holder (cat. no. 12501-13, Fine Science Tools)
- Extra-fine Graefe forceps, curved and 1 × 2 teeth (cat. no. 11155-10, Fine Science Tools)
- Extra-fine Graefe forceps, curved and serrated (cat. no. 11152-10, Fine Science Tools)
-
Surgical drape (cat. no. 28015-15, Fine Science Tools)
-
Gauze (Cutisoft; cat. no. 72223-05, BSN medical)
-
Cotton swabs (cat. no. 92811 BSN medical)
-
16-, 25-, and 27-G needle
-
1-ml and 20-ml syringes
-
Standard stereomicroscope with adjustable arm (M50, Leica)
-
Heating pad
-
Anesthesia inhalation tube
-
Anesthesia induction box
-
Animal hair clipper (epilatory cream can be used as alternative, but should be used with care as it can cause skin irritation)
-
Sutures: e.g., prolene suture 5-0 FS-3 needle, 45 cm (cat. no. 8614H, Ethicon)
Preparation of tumor cells
1.Thaw a cryovial containing 1-2 × 106 B16F10 cells (previously transduced with a lentiviral plasmid expressing a nuclear or cytoplasmic fluorophore) in 1 ml 10% DMSO/50% FBS/40% DMEM in a 37°C water bath. Quickly transfer cells to a 15-ml Falcon tube containing culture medium and centrifuge 3 min at 300 × g. Resuspend the cell pellet in 10 ml culture medium and transfer to a 100/20 mm culture dish.
2.Culture cells under standard growth conditions for the cell line, checking cell growth and morphology at least every 2-3 days.
3.When cells reach ∼80% confluency (typically after 2-3 days), wash them with 5 ml PBS and add 1-2 ml warmed trypsin/EDTA to detach the cells. After ∼3-4 min, inactivate the trypsin by adding 5 ml warmed culture medium. Split the cells in a ∼1:15 ratio for 1-2 weeks before the mouse injection. Incubate cells in a 37°C, 5% CO2 incubator.
4.To harvest the tumor cells, wash with 5 ml PBS and add 1-2 ml warmed trypsin/EDTA to detach the cells. After ∼3-4 min, inactivate the trypsin by adding 5 ml warmed culture medium. Collect the ∼6 ml of culture medium containing cells in a 15-ml Falcon tube and centrifuge 3 min at 300 × g , room temperature. Wash the cell pellet twice by resuspending the pellet in 5 ml PBS and centrifuging, and then repeating.
5.After resuspending the cell pellet in 5 ml PBS the second time, transfer 10 μl cell suspension to a dual-chamber cell counting slide. Count the cells using the automated cell counter; to ensure correct measurement, count the cells twice and take the average. Calculate the number of cells present in the 5-ml cell suspension.
6.Centrifuge the cell suspension 3 min at 300 × g , room temperature. Resuspend the cell pellet in the appropriate volume of PBS: For injection of 5 × 105 cells, the concentration of cells should be 5 × 106 cells/ml, of which 100 μl is used for injection. Therefore, resuspend the cell pellet in the appropriate amount of PBS to create the 5 × 106 cells/ml concentration. Transfer the cells to a 1.5-ml microcentrifuge tube and place it on ice.
7.Keep cells on ice until ready to inject them into mice. Inject as soon as possible, but at most 45-60 min after harvesting, to prevent cell death.
Preparation for surgery
8.Sterilize the surgical tools, surgical drape, gauze, and cotton swabs.
9.Using a 1-ml syringe with a 25-G needle, inject 100 μl of 0.03 mg/ml buprenorphine into the mouse subcutaneously (s.c.) for pain management 30 min before surgery.
10.Prepare the area of surgery by sterilizing surfaces with 70% EtOH.
11.Place a heat pad on the surgery bench and turn it on (it should be ∼37°C). Place anesthesia tube on the middle of the heating pad, and place a paper towel on top of heat pad to protect the mouse from overheating during the preparatory stages.
12.Place the adjustable brightfield microscope in such a way that you can shift the microscope over the field of view of surgery prior to the injection (see Fig. 1A). Clean the surfaces with 70% EtOH.
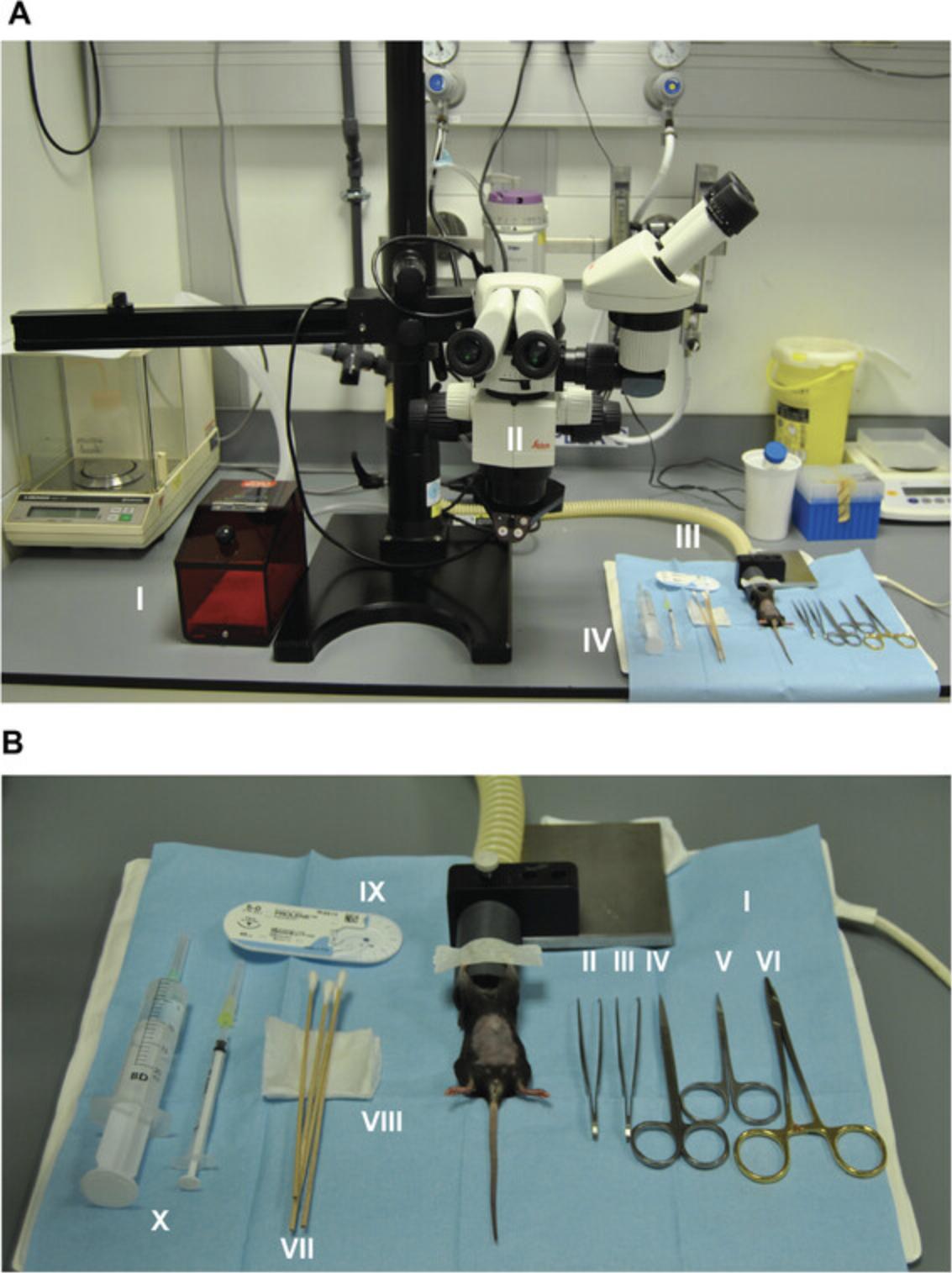
13.Weigh the mouse as a reference point for recovery after surgery.
14.Induce anesthesia of the mouse with 4% (v/v) isoflurane until the mouse does not respond with reflexes.
15.Transfer the mouse to the anesthesia tube placed on the heating pad. Continue anesthesia during surgery using 1%-1.5% (v/v) isoflurane, while monitoring the breathing patterns and reflexes of the mouse closely.
16.Apply ophthalmic ointment to the eyes of the mouse to prevent them from becoming dehydrated.
Mesenteric vein injection
17.Turn the mouse into supine position and carefully tape its front paws to the anesthetics tube (see Fig. 1B).
18.Using the animal razor, shave the abdomen and sterilize it using 70% EtOH
19.Place the sterile surgical drape under the mouse, and while maintaining sterility, place surgical tools, sutures, cotton swabs, gauze, 27-G needle, and 1-ml syringe on the surgical drape. Fill a 20-ml syringe with a 16-G needle with sterile PBS and place it on a surgical drape.
20.Start the incision in the skin using the fine scissors on the midline (caudal to cranial) and make a 15-mm incision (Fig. 2A). The incision should be placed in the middle of the animal's abdomen, 10-15 mm below the xiphoid. Using extra-fine Bonn scissors, continue the incision of the muscle wall. Use teethed extra-fine Graefe forceps to grab the muscle wall, make an incision, let go of the muscle wall, and grab it again more easily. In this way the pressure is released (Fig. 2B).
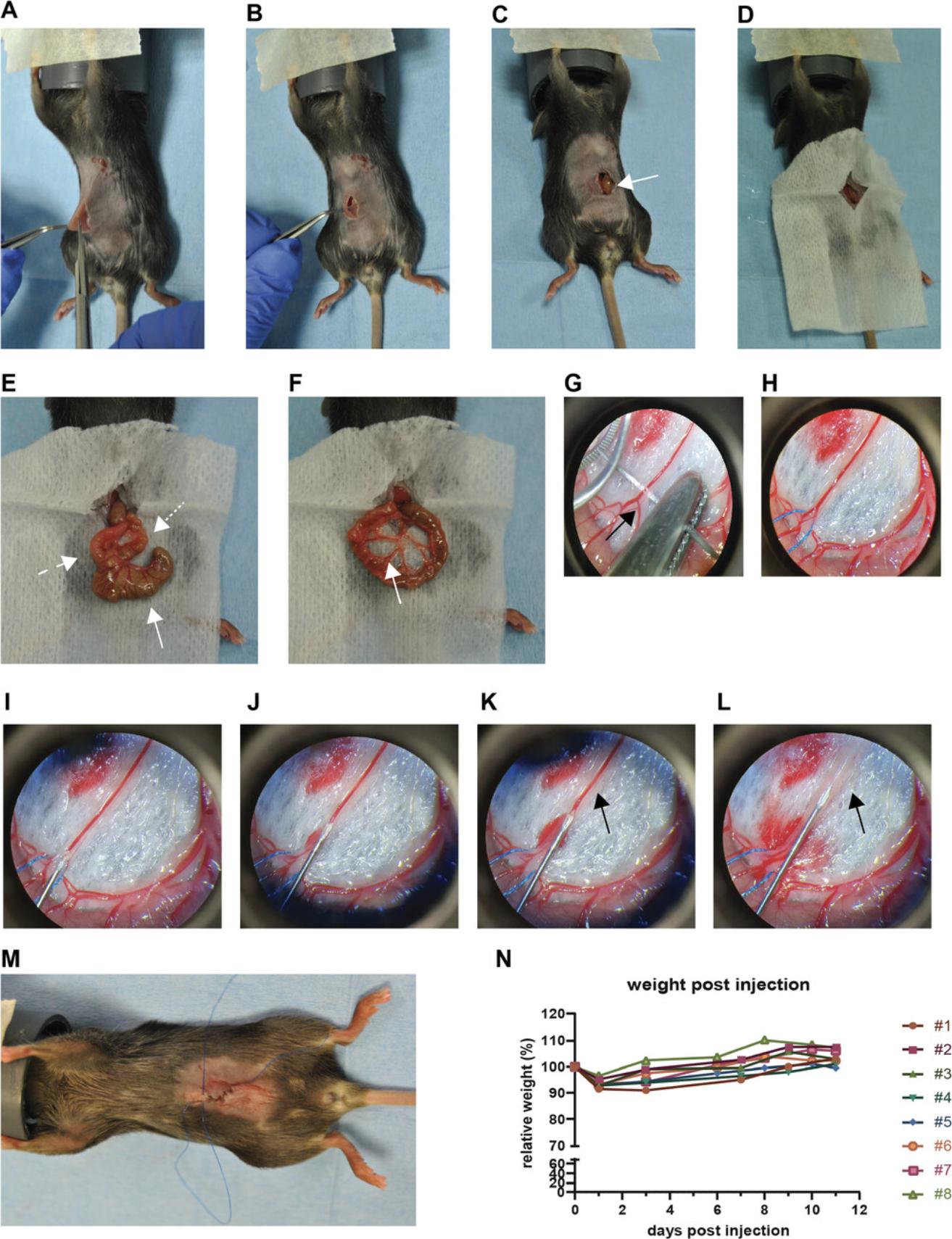
21.Using a cotton swab moistened with PBS and serrated forceps, locate the caecum and pull caecum toward the incision using the moistened cotton swabs (Fig. 2C; arrow points towards the exposed caecum).
22.Using the fine scissors, cut a small gap the size of the incision in the gauze. Place the gauze on top of the mouse, matching the gap to the incision (Fig. 2D).
23.Using two cotton swabs moistened with PBS, carefully pull the caecum out of the abdomen and place it on the gauze (Fig. 2E).
24.Locate the small intestine and pull it slightly out of the abdomen.
25.Place the caecum back in the abdomen, leaving the small intestine out.
26.Spread the small intestine and its mesentery on the moistened part of the gauze (Fig. 2F).
27.Locate a merging of mesentery vessels into one vessel (indicated with an arrow in Fig. 2F and H).
28.Place the adjustable stereomicroscope over the exposed small intestine and mesenteric vein to help with the precision of the injection.
29.Place the suture underneath the intersection of the two vessels for stability during the injection (Fig. 2G and H).
30.Clamp the ends of the stitch ∼5 cm below the stitch using the Halsey needle holder.
31.Resuspend the cancer cells in PBS using a 1-ml pipet. Prepare the tumor cells in PBS in a 1-ml syringe and place a 27-G needle on the syringe. Adjust the volume in the needle to 100 μl.
32.While holding tension on the stitch keeping the mesenteric vein in place, carefully insert the needle at the intersection of the two mesenteric veins into the extending vein until the needle opening is inside (Fig. 2I).
33.Slowly, over ∼10 s, inject 100 μl tumor cells in PBS in the mesenteric vein.
34.Press the vein closed with a dry cotton swab and carefully retract the needle. Press vein closed for ∼2 min, or until bleeding has stopped.
35.Flush the mesentery around the injection site with sterile PBS to wash away potential remaining tumor cells. Tap the area dry with a piece of gauze and repeat this at least twice.
36.Carefully place the small intestine and mesentery back in abdomen using PBS-wetted cotton tips.
37a. When preparing for long-term, multiday intravital imaging: Continue to step 3 of Basic Protocol 3.
37b. When preparing for short-term intravital imaging at a later timepoint: Close the muscle wall and skin using an uninterrupted running or continuous suture (Kudur, Pai, Sripathi, & Prabhu, 2009; Fig. 2M). Continue to step 38 below.
Post-injection monitoring of mouse
38.Place the mouse back in its cage, place half of the cage on the heating pad for a better recovery, and closely monitor the mouse's recovery and wellbeing after the surgery by checking mobility and appearance (Te Velde et al., 2003).
39.The next day and every 2-3 days after, monitor the mouse after surgery by measuring it weight, behavior, appearance, and mobility as described by te Velde et al. (te Velde et al., 2003).
Basic Protocol 2: SHORT-TERM IMAGING OF TUMOR CELLS IN MOUSE LIVER
At a chosen timepoint after mesenteric vein injection, the liver can be used for intravital imaging at an endpoint imaging session. The mouse liver can be imaged once, either directly after mesenteric injection or days to weeks later, using an upright multiphoton stage. After the imaging session, the mouse should be euthanized and the liver can be harvested for further analysis, such as immunohistochemistry or protein or gene expression analysis. This protocol is suited to examining short-term behavior of tumor cells in the liver. Imaging can be done from <1 hr up to 8 hr.
Materials
-
Mice following mesenteric vein injection of tumor cells (Basic Protocol 1)
-
100% (v/v) isoflurane
-
Sterile PBS
-
Upright multiphoton microscopy system (in this protocol, we used a Zeiss LSM710 NLO upright multiphoton equipped with a Mai Tai DeepSee multiphoton laser (690-1040 nm) and W Plan-Apochromat 20×/1.0DIX M27/75 mm objective)
-
Breathing monitor, optional
-
DAKO pen (cat. no. S200230-2, Agilent Technologies)
-
Custom microscope insert including heated pad providing heating feedback to regulate and maintain the temperature of the mouse (Fig. 3C, H, and I; a rectal probe with heating feedback system can be used instead of the heating pad)
-
Anesthetic induction box
-
Anesthetic inhalation tube
-
Surgical tools:
- Fine scissors (cat. no. 91460-11, Fine Science Tools)
- Extra-fine Graefe forceps, curved and 1 × 2 teeth (cat. no. 11155-10, Fine Science Tools)
- Extra-fine Graefe forceps, curved and serrated (cat. no. 11152-10, Fine Science Tools)
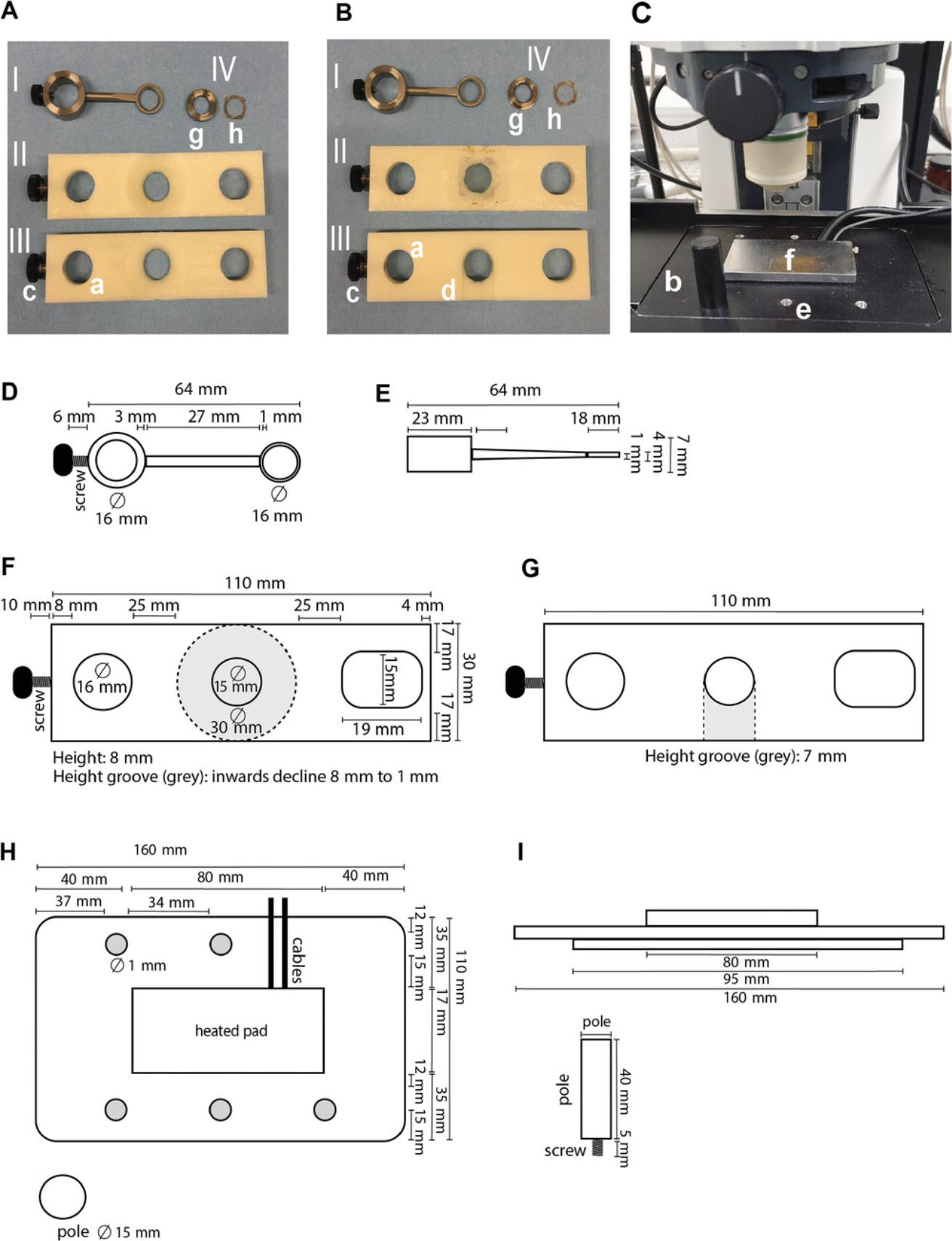
- Cotton swab (cat. no. 92811, BSN Medical)
- Gauze (Cutisoft; cat. no. 72223-05, BSN Medical)
- Tissue glue (Histoacryl®; cat. no. 1050052, Braun)
- Tape
- Custom titanium coverglass holder (Fig. 3A and 3B, I and II, and Fig. 3D and E)
- 12-mm no. 1.5 coverglass (Menzel, cat. no. MENZCB00120RAC20)
- Fiji software (Schindelin, Arganda-Carreras, & Frise, 2012)
Preparation of the mouse
1.Turn on the microscope system, laser, and software.
2.Turn on the breathing monitor (optional) and heating pad (Fig. 3C, H, and I).
3.Induce anesthesia of the mouse under 4% (v/v) isoflurane in the anesthesia induction box until the animal does not respond to pain reflexes.
4.Move the animal onto the microscope platform with heating pad in supine position, and place with its head in an anesthesia tube (Fig. 4A). Lower isoflurane anesthesia to ∼1% (v/v). Carefully monitor the breathing and pain reflexes to ensure proper anesthesia.
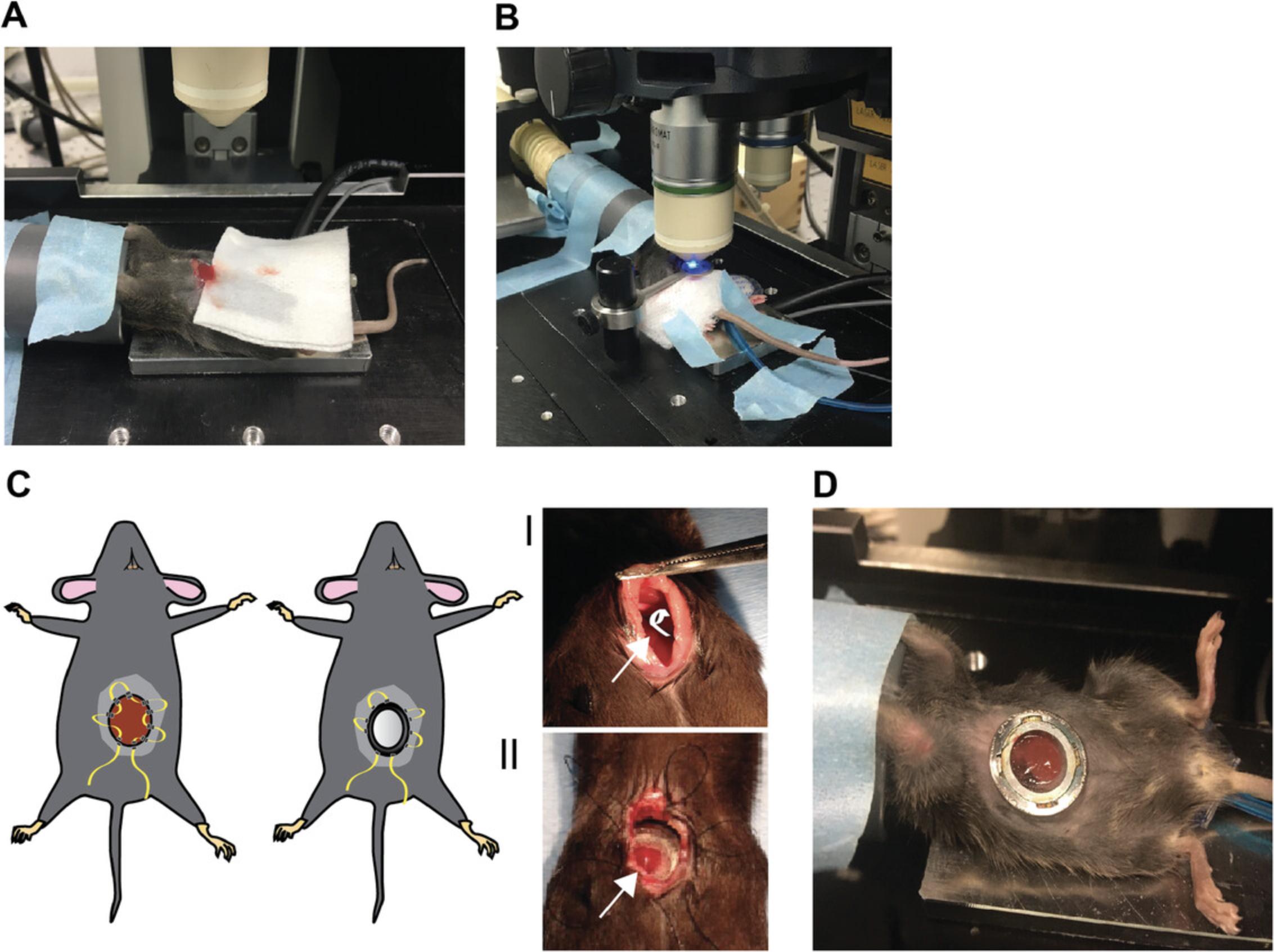
5.Using the fine scissors, cut the skin and muscle wall using a 10- to 15-mm horizontal incision 5 mm below the right ribcage, starting at the midline.
6.Using moistened cotton swabs, locate the liver lobes. For imaging, the left lobe is most preferred.
7.Carefully pull and position the left lobe so that it is placed frontally (Fig. 4A).
8.Place a piece of gauze under the liver lobe, and use tissue glue to carefully glue the edges of the lobe to the gauze (Fig. 4A).
9.Carefully exteriorize the liver lobe partially out of the abdomen to prevent movement of the lobe during imaging. Using tape, secure the gauze in place to prevent retraction during imaging (see Fig. 4B).
10.Carefully press the titanium or plastic coverglass holder, including coverglass (Fig. 3A and B, I and II), on top of the liver. Circle the outline of the coverglass with the DAKO pen. After the DAKO pen mark dries, add a droplet of water to allow visualization through the microscope.
General intravital imaging setup
11.To determine whether the liver lobe contains tumor cells suited for imaging, place the mouse under the objective and look through the eye pieces.
-
We recommend using a ≥20× objective to be able to properly identify the tumor cells.
-
Blood vasculature will appear dark, whereas the liver tissue will appear fluorescent due to autofluorescence (Fig.5A).
-
The fluorescent labeling of the tumor cells should be visible.
-
Hairs are autofluorescent and have a very bright, distinct pattern (Fig.5B). As light does not pass through the hair, imaging deeper results in a black streak in the imaging frame.
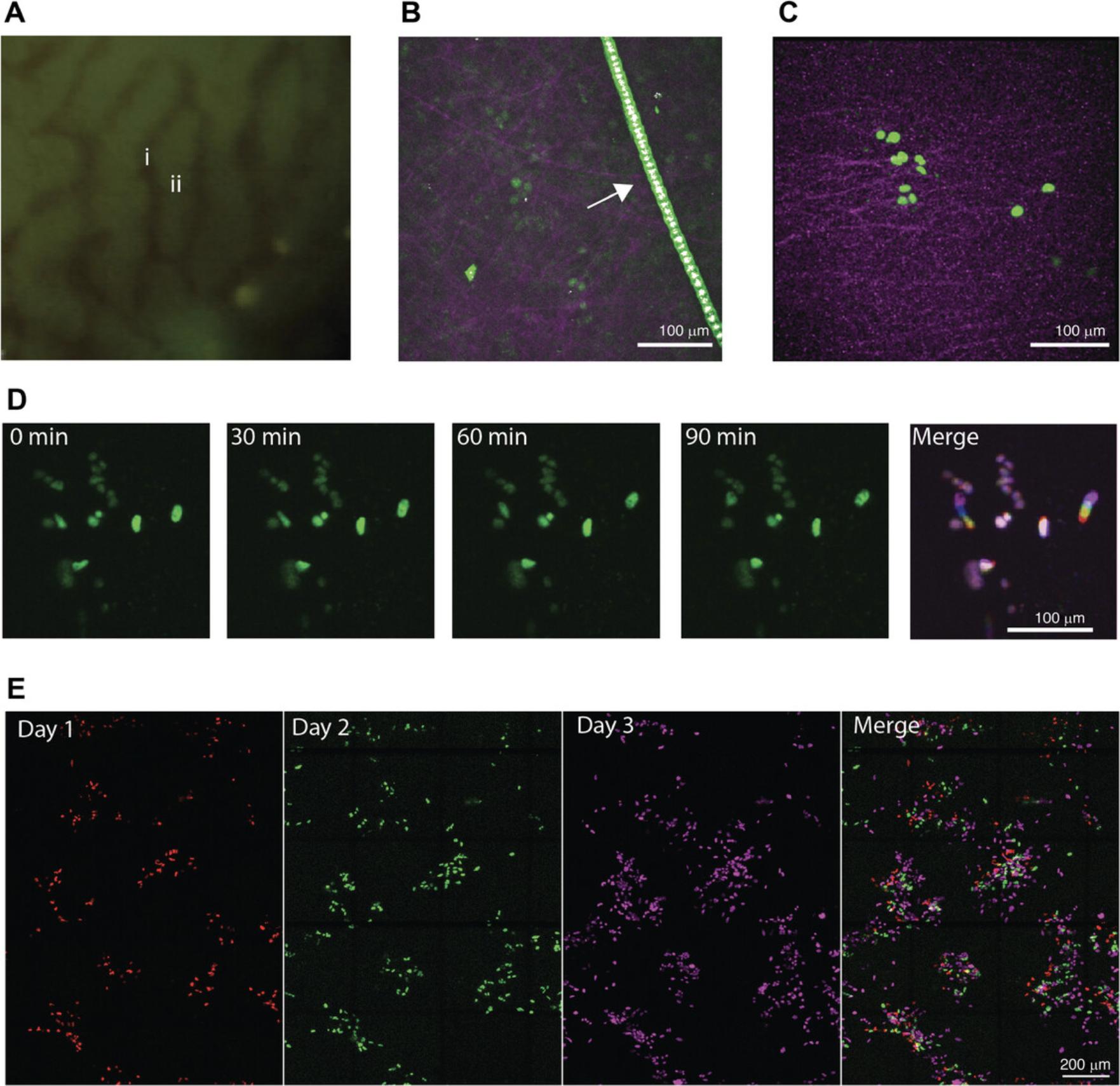
12.The pinhole can be completely opened to collect as much light as possible, as optical sectioning is inherent to multiphoton microscopy and does not require a pinhole.
13.To enhance speed of imaging and thereby minimize breathing artefacts, we recommend acquisition in 512 × 512 pixels (image size of 425.10 μm × 425.10 μm) and bidirectional scanning.
14.As there are often large differences between signal intensities, especially when imaging z stacks, imaging in 12-bit format is preferred.
15.Settings such as imaging speed and line averaging can be adjusted to achieve a favorable interval between images. Averaging based on line scan is preferentially used to improve image quality, as otherwise movement in between the averaging can result in unsharp objects. A line average of 2 is used in this protocol.
16.Depending on the signal of the fluorophore and the excitation wavelength, laser power between 3% and 10% can be used. With some multiphoton lasers, the output intensity is not equal when comparing different wavelengths. In our case, above 900 nm the output drops, so a power between 10% and 15% can be used, whereas at <850 nm it is recommended that the laser power remain under 10% to avoid tissue damage.
17.When unwanted movement is introduced during imaging, due to breathing of the animal or traction of the tissue, registration plug-ins such as “descriptor-based series registration (2d/3d + t)” in Fiji can be used to correct this movement in 4D stacks based on the signal of the tumor cells or the second-harmonic generation (SHG).
Short-term intravital microscopy
18.If hair is visible in the imaging frame, rinse the liver carefully with PBS.
19.For short-term imaging, recording separate positions is sufficient. We recommend taking 3-5 field of views per imaging session, depending on the amount of visible tumor cells.
20.We recommend total imaging times between 1 and 4 hr, but extending imaging times up to 8 hr is possible.
21.For precise migration analysis, a frequency of 10-15 min between frames is ideal; however, this can be dependent on the migration speed of the cell line (Chen, Ritsma, & Vrisekoop, 2019). To measure migration in 3D, use a z stack with a step size between 1 and 2 μm.
22.After imaging, the animal can be sacrificed using cervical dislocation or CO2 inhalation. Organs can be harvested for further analysis.
Basic Protocol 3: LONG-TERM IMAGING OF TUMOR CELLS IN MOUSE LIVER USING AN ABDOMINAL IMAGING WINDOW
This protocol is an improved adaptation of the abdominal imaging window (AIW) developed by Ritsma et al. (Ritsma et al., 2013; 2012). In addition, the protocol presented here describes imaging using an upright multiphoton stage. This protocol adds to Basic Protocol 1 through the implantation of an AIW after the mesenteric vein injection to allow multiday intravital imaging. Perform Basic Protocol 1 until step 37a and then proceed with Basic Protocol 3.Note that at step 19, the incision should be ∼15 mm and end 3 mm over the xiphoid, instead of 10 mm under the xiphoid. For proper implantation of the AIW, two persons are needed.
Materials
-
70% ethanol (EtOH), for sterilization
-
Sterile phosphate-buffered saline (PBS)
-
100% (v/v) isoflurane
-
Ophthalmic ointment (Oculentum Simplex 5 g, sterile; cat. no. 220201, Added Pharma)
-
Abdominal imaging window (AIW) holder (Fig. 3A and B, III, and Fig. 3F and G)
-
12-mm no. 1.5 coverglass (Menzel, MENZCB00120RAC20)
-
Cyanoacrylate glue (Super Gel, Pattex)
-
Custom-made AIW and removable window insert (Fig. 3A and B, IV, g and h)
-
DAKO pen (cat. no. S200230-2, Agilent Technologies)
-
Surgical tools:
- Extra-fine Bonn scissors (cat. no. 14084-08, Fine Science Tools)
- Fine scissors (cat. no. 91460-11, Fine Science Tools)
- Halsey needle holder (cat. no. 12501-13, Fine Science Tools)
- Extra-fine Graefe forceps, curved and 1 × 2 teeth (cat. no. 11155-10, Fine Science Tools)
- Extra-fine Graefe forceps, curved and serrated (cat. no. 11152-10, Fine Science Tools)
- Hartman hemostat (cat. no. 13002-10, Fine Science Tools)
- Ring forceps (cat. no. 11103-09, Fine Science Tools)
- Dumont no. 5 forceps (cat. no. 11252-40, Fine Science Tools)
-
Gauze (Cutisoft; cat. no. 72223-05, BSN medical)
-
Surgical drape (cat. no. 28015-15, Fine Science Tools)
-
Cotton swabs (cat. no. 92811 BSN Medical)
-
Sutures (prolene suture 5-0 FS-3 needle, 45 cm; cat. no. 8614H, Ethicon)
-
Heating pad
-
Anesthesia inhalation tube
-
Anesthesia induction box
-
Upright multiphoton microscopy system (in this protocol, we used a Zeiss LSM710 NLO upright multiphoton equipped with a Mai Tai DeepSee multiphoton laser (690-1040 nm) and W Plan-Apochromat 20×/1.0DIX M27/75 mm objective)
-
Custom microscope insert including heated pad providing heating feedback to regulate and maintain the temperature of the mouse (Fig. 3C, H, and I; a rectal probe with heating feedback system can be used instead of the heating pad)
Preparation of abdominal imaging window
1.Glue a coverglass on the outer side of the AIW insert (Fig. 3B, IV, h) using cyanoacrylate glue. Carefully press the coverglass onto the window insert. Let the insert dry >12 hr.
2.Place the window insert (Fig. 3A and 3B, h) in the AIW (Fig. 3A and 3B, g) to finalize the AIW. To sterilize, leave the AIW containing the insert in 70% EtOH for >30 min prior to surgery. Allow the imaging window to dry before continuing with step 3.
Implantation of abdominal imaging window
Perform AIW implantation after tumor cell injection (Basic Protocol 1, steps 17-37a).
3.Clamp 50 mm of the xiphoid using the Hartman hemostat. Leave the xiphoid clamped up to step 8.
4.Lift the Hartman hemostat in cranioventral direction. With a moistened cotton swab, gently press the liver posterior to visualize the falciform ligament. Using extra-fine Bonn scissors, carefully dissect the falciform ligament, being careful not to puncture the diaphragm.
5.Using fine scissors, cut a piece of sterile cotton gauze in 25 × 10 mm. Using the Dumont no. 5 forceps, roll up the 25 × 10-mm cotton gauze around it, and then slide it off. Moisten the roll with sterile PBS.
6.Using moistened cotton swabs and the ring forceps, move the right liver lobes anteriorly and laterally, exposing the left liver lobe. With serrated Graefe forceps, place the cotton roll behind the left liver lobe and the diaphragm to push the lobe caudally and upwards (Fig. 4C, Il; arrow points to the location of the cotton roll, shown in white in the drawing at left).
7.Briefly fit the window into the incision to ensure the incision is of the proper size. Remove and set aside. Manipulate the left liver lobe so that it is placed behind the incision. Apply glue to the interior ring surface of the AIW (Fig. 3B, IV, g) using the back of a cotton swab or a tissue tip. Lift the incision on one side using extra-fine Graefe forceps, place the window on the liver in one movement, and gently apply pressure for a few minutes. Allow the glue to dry for ∼5 min.
8.Remove the Hartman hemostat from the xiphoid. Dissect the clamped tissue using fine scissors.
9.While the glue is drying, proceed with applying a purse-string suture around the incision (Fig. 4C). To do this, start at the right caudal end of the incision. For the first stitch, pass the suture thread from outside (skin) to inside (abdominal wall) 2 mm away from the incision line, and re-insert from inside to outside 5 mm away from the first insertion. Repeat this pattern by re-inserting the suture thread from outside to inside, creating a loop with the thread about two loops on the right side and two loops on the left side of the incision. The purse-string suture will end on the left caudal side of the incision, 5 mm from the start of the suture. Leave the suture loops untightened until step 10.
10.Using serrated forceps, carefully insert the skin/muscle wall with suture into the groove of the ring by carefully lifting up the window (Fig. 3B, g). Once the window is fitted into the groove, pull the loops one by one, starting with the most cranial loop. Finish the suture by pulling the caudal left and right suture end, and secure the suture with an instrument tie (surgeon's knot).
11.Place the mouse in prone position to allow settling of the liver and abdominal organs.
12.Place the mouse back into the cage for recovery on a heating pad. Monitor recovery after surgery.
13.When imaging with an upright microscope, it is advisable to start imaging 1 day after AIW surgery to allow proper settlement of the liver to the window.
Multiday multiphoton imaging
See also general notes for intravital microscopy settings in Basic Protocol 2, steps 11-17.
14.Induce anesthesia of the mouse under 4% (v/v) isoflurane in the anesthesia induction box until the animal does not respond to pain reflexes.
15.Move the animal onto the microscope platform in supine position, and place with its head in an anesthesia tube (Fig. 4D). Lower isoflurane anesthesia to <1% (v/v). Carefully monitor the breathing and pain reflexes to ensure proper anesthesia.
16.Apply ophthalmic ointment to prevent drying of the eyes
17.Place the window in the window holder (Fig. 3A and B, III) and place the window in the groove. Circle the outline of the coverglass with the DAKO pen. After the DAKO pen mark dries, add a droplet of water to allow visualization through the microscope. Locate tumor cells through the ocular.
18.Once tumor cells are located, imaging sessions can start.
19.Imaging sessions can be performed up to 2 weeks, with a max of ∼5 imaging sessions, giving animals >24 hr breaks for proper recovery.
20.Separate positions, or a larger tile scan can be scanned during one imaging session, keeping the duration of the scan between 45 min and 1 hr.
21.Individual tumor cells or clusters can be traced back either by identifying tumor cell clusters or by using SHG signal that is generated by collagen-1 fibers (Brown et al., 2003).
22.During longer intravital microscopy experiments, exudate can form on the inside of the coverglass. In a sterile environment, twist out the ring with coverglass and remove the exudate. If the ring is stuck, incubate the window and ring with PBS to dissolve the exudate which is stuck in the insert. Then flush the liver with sterile PBS and place the coverglass and ring back in the window.
23.After imaging, place the mouse back in the cage. It should recover within 5 min.
24.After the last imaging session, the animal can be sacrificed using cervical dislocation or CO2 inhalation. Organs can be harvested for further analysis. The abdominal imaging window can be removed, cleaned, and sterilized for reuse.
COMMENTARY
Background Information
The liver is a clinically relevant organ for metastasis. In a study of 4,399 patients with adenocarcinoma, liver metastasis was the second most prevalent metastatic site, and 41% of metastases were found in the liver (Hess et al., 2006). Circulating tumor cells that arrive in the liver arrest in the vasculature of the hepatic sinusoids because of low blood flow. Due to the proinflammatory environment of the liver, the majority of tumor cells will be eliminated. Some tumor cells, however, will have the ability to evade or resist hepatic defense mechanisms and adhere to sinusoidal endothelium and undergo extravasation. To promote tumor cell survival and growth, a supportive niche is recruited, containing (activated) tumor-supportive stromal cells such as activated hepatic stellate cells and hepatocytes. This niche supports angiogenesis to enable metastatic outgrowth beyond a 300-μm size. These individual phases of liver colonization have been described in detail by others (Brodt, 2016; Marvin et al., 2020; Vidal-Vanaclocha, 2008). As illustrated by Vidal-Vanaclocha and Brodt (2008), the tumor microenvironment plays a key role in the development of liver metastasis and has distinct functions in each phase. This highlights the need to study these processes in their biological microenvironment. Despite advances with ex vivo models of the liver microenvironment, the current models do not recapitulate the full microenvironment of liver metastases (Clark, Ma, Taylor, Griffith, & Wells, 2016).
Mouse models of spontaneous metastasis can be used to study liver metastasis; Gomez-Cuadrado et al. provide an overview of commonly used (spontaneous) mouse metastasis models and metastatic colonization to multiple organs, including the liver (Gomez-Cuadrado et al., 2017). However, although these models can reflect the full metastatic cascade, they are of limited use for intravital microscopy experiments because of the unpredictable speed of spontaneous metastasis to the liver, the low frequency of metastasis, and the unpredictability of where in the organ the metastasis will form—a concern given that only the surface (outer 1 mm) of the (left) liver lobe can be imaged using multiphoton intravital imaging. By contrast, experimentally induced liver metastasis can result in a robust, widespread metastatic pattern to the liver, providing additional control over the timing and resulting in more homogenously sized metastases. This leads to more reproducible results and a reduction in the number of mice needed.
A commonly used approach to induce liver metastasis is by injecting murine tumor cells intrasplenically (Ritsma et al., 2012; Soares et al., 2014; Zhang & Du, 2019). This results in efficient liver metastasis formation but has a major drawback: Because of the suitable environment of the spleen, tumor cells remaining after injection can efficiently form a splenic tumor. This necessitates splenectomy, which is suboptimal because it severely impacts the immune system (Higashijima et al., 2009). As the current knowledge of the effects of the immune system during (liver) metastasis formation is far from complete, and considering the recent clinical successes with immunotherapies in cancer, it is highly beneficial to maintain a functional immune system in mouse models to be able to study its contribution. Therefore, as an alternative to intrasplenic injection, portal vein injections can be used to induce liver metastasis (as described by Goddard, Fischer, & Schedin, 2016). Although this approach leads to efficient injection, it can be technically challenging. In particular, due to the larger size of the portal vein, it can result in excessive bleeding after tumor cell injection. A mortality rate of 30% was reported for this technique (Goddard et al., 2016), which could be improved to 2% using a piece of hemostatic gauze. In addition, due to the high bleeding risk, a smaller needle (32-G) must be used, which can result in increased sheer stress damage to the cancer cells.
With these protocols, we provide another alternative approach to creating liver metastases that involves injecting murine tumor cells into one of the mesenteric veins, which are located in the mesentery of the small intestine. Mesenteric vein injection leads to fewer complications and does not affect the murine immune system. To apply this approach in an immunocompetent host requires injecting murine cancer cells. However, a similar approach can be used to inject human cancer cells in immunodeficient mice (Kuo et al., 1993). To retain parts of the immune system in that case, a humanized mouse model could potentially be used (Allen et al., 2019; Huey & Niewiesk, 2018).
Although methods such as histological staining of sections and gene expression profiling of (primary and metastatic) cancer cells have resulted in numerous insights into (liver) metastasis, they provide only snapshots in time of this highly dynamic process (Auguste et al., 2007; Yamasaki et al., 2007). By using multiphoton imaging, dynamic processes can be studied at subcellular resolution in their biologically relevant environment. Additional information can be obtained during imaging by visualizing parts of the microenvironment, such as the collagen-1 fibers through second-harmonic generation (SHG), the vascular system using fluorescently labeled dextrans, and immune cell subsets using specific fluorescent antibodies (Headley et al., 2016; Ritsma et al., 2012). Moreover, molecular signaling dynamics in the tumor cells can be visualized using fluorescent reporters (Giampieri, Pinner, & Sahai, 2010; Matsuda & Terai, 2020; Muta et al., 2018). Through this approach, alterations in signaling pathways and cellular behavior can be studied at high resolution, in vivo, over time, providing unique insights into the dynamic metastatic process as well as responses to therapies (Margarido, Bornes, Vennin, & van Rheenen, 2019). For example, Ritsma et al. showed that C26 colorectal cancer cells form pre-micrometastases after extravasation to the liver, where cells displayed higher motility, before forming less migratory micrometastasis. Suppressing tumor cell migration in this pre-micrometastatic phase resulted in a reduced metastatic load (Ritsma et al., 2012). Fumagalli et al. used intravital microscopy to visualize colorectal cancer primary tumors and liver metastases, and demonstrated cellular plasticity during the metastatic cascade (Fumagalli et al., 2020). Migratory cells that escaped the primary tumor were mainly Lgr5 deficient, while intravital imaging showed that Lgr5 was re-expressed in liver metastasis. Using this approach, the authors showed that stem cell plasticity is necessary for metastatic seeding in the liver. In addition, intravital imaging has been used to obtain information about mechanisms of therapy resistance, such as resistance to mitogen-activated protein kinase kinase and BRAF small-molecule inhibitors in melanoma (Brighton et al., 2018; Hirata et al., 2015) and tumor microtube-induced resistance to conventional therapy in glioblastoma (Weil et al., 2017). Moreover, it provided insights about the consequences of administration of therapeutic agents, for example the increased risk of metastatic dissemination of breast cancer cells upon neoadjuvant treatment (Karagiannis et al., 2017).
The approach presented here combines the robust induction of experimental liver metastasis using mesenteric vein injection with short- or long-term intravital imaging. Using this approach, migration and outgrowth dynamics of liver metastases of different cancer types can be studied in a biologically relevant mouse model. This will enable novel discoveries targeting hepatic colonization of metastatic tumor cells.
Critical Parameters
Sterile work environments for cell culture and surgeries
For optimal viability of cancer cells and health of the mice, it is essential to work in a clean and sterile environment, using sterile solutions and tools (Pritchett-Corning, Mulder, Luo, & White, 2010; Stacey, 2011). Because not all infections in cell culture are visible by eye, it is advisable to perform regular mycoplasma testing as well as a mouse and rat antibody production (MAP/RAP) test prior to mesenteric vein injections. In vivo, infections can affect the growth of the tumor cells in the liver, as well as the overall well-being of the animal. Infection in vivo can present itself as worsened behavior of the animal based on the clinical appearance score (Te Velde et al., 2003), but also as an enlarged/swollen abdomen. In the abdominal imaging window, an infection appears as a cloudy white layer behind the window. Minor infections can be treated with antibiotics; however, these might affect metastasis formation (Lamb et al., 2015). Most infections will ultimately lead to a decision to sacrifice the animal. To minimize contamination, it is important to properly autoclave surgical tools, gauze, cotton swabs, and surgical drapes, as well disinfecting the surgical area and hands.
Cell culture
For all cell culture practices, the variability between cell lines can lead to heterogeneity between experiments (Ben-David et al., 2018; Liu et al., 2019). We strongly recommend creating and freezing a large stock of cryovials with frozen cells shortly after generating the required cell line and then thawing individual cryovials before each experiment. Close monitoring of the morphology and proliferation speed of the generated cell line and thawed cell line is advised; these should be compared to those of the parental cell line. In the case of B16F10, variation can occur quickly, and the level of pigmentation should also be monitored. Cell authentication to confirm the identity of the cell line is recommended, and commercially available profiling kits for mouse cells, such as the ATCC®'s mouse short tandem repeat profiling, can be used.
Mesenteric vein injection
Choosing the experimental mouse conditions
In this protocol, immunocompetent mice are employed in combination with syngeneic murine cancer cells. Different mouse strains can be used as long there is a syngeneic match with the murine cancer cell. Mice between 8 and 12 weeks of age are best suited for experimentation (Speak et al., 2017). Older mice (10-12 weeks) are preferred for window implantation due to their larger anatomical structures and increased weight. It is preferable to use female mice because of their less aggressive nature. Moreover, Speak et al. showed preferential tumor growth in female mice (Speak et al., 2017). However, certain cancer types, e.g., prostate cancer and breast cancer, can require male or female mice specifically.
Misinjection and choosing the right mesenteric vein
For optimal metastatic outgrowth of tumor cells, it is important to keep the cells on ice and inject them as quickly as possible after collection. One problem that can occur is tumor outgrowth in the mesentery, which is caused by mis-injection or by tumor cells that remain after insufficient flushing following injection. After the mice are sacrificed, this should always be checked. The selection of a good mesenteric vein to inject can help prevent mis-injections; in particular, the presence of overlaying vessels often results in excessive bleeding and mis-injection, and very small vessels are harder to inject. In younger mice (8 weeks), higher-branching vessels can be used for injection, due to the smaller size and decreased likelihood of bleeding. In older mice (>10 weeks), however, it is advisable to use the smaller branching veins that are located closer to the intestine to prevent excessive bleeding. During correct injection, the blood of the vessel will be replaced by the colorless PBS. In the case of a mis-injection, the mesentery around the vessel will swell. To prevent leakage of the injected tumor cells, it is advisable to keep the needle in the vein for a few additional seconds after the injection is finished, to allow the cells to travel to the liver. Ideally, regular blood flow will replace the PBS at this point. When retracting the needle, a cotton swab can be used to immediately press on the vein; this helps prevent leakage and reduce bleeding.
Preventing damage to small intestine
Because the mesenteric vein injection is performed with exposed small intestine, and partially under the light of a stereomicroscope, it is important to prevent drying of the intestines. After exposing the intestine, make sure to regularly (e.g., every 3 min) moisten the intestine and mesentery with PBS. Additionally, handle the intestines carefully using moistened cotton swabs, and expose as little intestine as possible during the injection.
Implantation of abdominal imaging window
Proper placement of liver and preventing movement of liver tissue
To prevent movement of the liver during imaging, it is essential that the falciform ligament is properly cleaved. This can be achieved by gently pulling the liver downward using a moistened cotton swab, while simultaneously opening the abdominal cavity by elevating the xiphoid. The ligaments can be dissected in a pulling motion. This must be done with extreme care to avoid puncturing the diaphragm or lungs, resulting in death. For proper placement of the liver, the gauze roll should be placed behind the left lobe of the liver. Correct placement will take practice and is key for successful window implantation. For a correct placement, the liver lobe should be pressed caudally and upward, so that it is visible underneath the incision without being held in place with a cotton swab. Liver should be handled with moistened cotton swabs. To prevent autofluorescent hair from sticking to the liver, it can be flushed with sterile PBS.
Correct window implantation
The experimental procedure for proper window placement requires two people. Before implantation, ensure that the incision is big enough to fit the window and faces the correct lobe. Seconds before implantation, glue should be applied on the bottom side of the window. The glue cannot be old and should be stored at 4°C. Old glue will result in the release of the window from the liver, either directly or within the next 24 hr. Once the window is placed, it cannot be removed; this will result in liver tissue damage. After placing the window, it is important not to manipulate the window for at least 5 min to ensure its proper fixation.
Intravital imaging
Surgical exposure
For good intravital imaging recordings, it is essential that movements due to breathing of the mice are minimized. This can be done using tape and tissue glue, while preventing damage to the liver tissue. Moreover, keeping the liver moistened with PBS will prevent movement of the liver itself. Placing a moistened piece of gauze on exposed tissue that will not be imaged can help to prevent the liver from drying out. The frequency of imaging is dependent on the process that is to be imaged.
Imaging through abdominal imaging window
When too many cells are present in the field of view due to the injection of too many cells, or to excess growth, individual cells cannot be traced over long periods of time using the window. This problem can be solved by imaging at earlier timepoints, injecting fewer cells, or labeling a few cells with photoconvertible Dendra (Gligorijevic, Kedrin, Segall, Condeelis, & van Rheenen, 2009).
Choice of fluorophore
For short-term intravital imaging over sequential timepoints, simultaneous excitation of the fluorophores is required. When using multiple fluorophores, it is necessary to make sure that they can be properly excited using the same wavelength on the multiphoton microscope (Drobizhev, Makarov, Tillo, Hughes, & Rebane, 2011).
SHG signal can be produced by collagen-I fibers during excitation of tumor cells, which results in the generation of photons with a wavelength half the emission wavelength (Brown et al., 2003). To detect the SHG, it is necessary to have a filter present that can detect half the wavelength of your expected emission wavelength. This can alter the choice of fluorophore used for visualizing the tumor cells. Of note, not all collagen-I can or will generate SHG, so lack of signal does not mean a lack of collagen-I.
Understanding Results
In our work, we transduced B16F10 cells with the lentiviral vector pLenti containing a silencing-prone spleen focus-forming virus (SFFV) promoter driving a H2B-mTurquoise fluorophore using a standard transduction protocol. The vector and transduction protocol are available from the corresponding author upon request. Four days after mesenteric vein injection, the cells were imaged using surgical exposure (Fig. 5C). mTurquoise was excited at 855 nm, resulting in the visibility of the tumor cells (green) and SHG generated by collagen-I fibers (pink), emitted at 427 nm. At this early timepoint after injection, small clusters of tumor cells are visible. The collagen-I fibers can be used as a reference point to align the sequential images. A smoothened maximum projection of a z stack of 42 μm, with 1.5-μm steps, is shown (Fig. 5C). In a different animal, small clusters of B16F10 cells expressing H2B-mTurqoise were excited at 855 nm, and the tumor cell signal appeared in green (Fig. 5D). Tumor cells were imaged over time at intervals of 10 min; timepoints at 0, 30, 60, and 90 min are shown. A maximum projection of a z stack is shown. A merge of the different timepoints, false-colored with different colors, shows non-moving cells in purple and moving cells with a rainbow trail.
D2.A1 cells (obtained from the Karmanos Cancer Insitute, Detroit, MI, USA) were transduced with the lentiviral vector pLV vector containing a CMV promoter driving the expression of the fluorophore Dendra2. The vector and transduction protocol are available from the corresponding author upon request. D2.A1 cells expressing H2B-Dendra were imaged using the AIW at 1 (red), 2 (green) and 3 (pink) days post injection (Fig. 5E). Cells are differently false colored on the various days to enable better visualization of the presence of the cells at the various days in the overlay. Cells were excited at 960 nm. A large overview tile scan, consisting of multiple merged tiles, was made every day, and a maximum projection of the recorded z stack is shown. The position of the tumor cell clusters and SHG signal (not shown) were used to align the images at different timepoints to visualize the tumor cell migration and proliferation (merge). White spots indicate a merged signal for days 1, 2, and 3, whereas the individual colors show tumor cells that are at different location at the different timepoints.
Troubleshooting
Table 1 lists problems that may arise with this procedure as well as their possible causes and solutions.
Problem | Possible cause | Solution |
---|---|---|
Tumor in the mesentery No tumor cells visible |
Mis-injection of tumor cells, or tumor cells remaining after injection | Improve injection technique; ensure proper flushing after injection. |
Tumor cells did not take because of reduced viability of tumor cells prior to injection | Decrease the time between collecting cells and tumor cell injection. Also, make sure not to let tumor cells pass through the needle twice; take up tumor cells in the syringe without a needle or using a large (<18-G) needle. | |
A longer time is needed for metastasis formation | Perform exploratory (non-intravital imaging) experiments and examine metastasis by immunohistochemistry at different timepoints after injection. | |
Metastases are not formed behind the AIW, but in a different location in the liver | Inject more tumor cells to increase the chance of metastasis formation under the AIW. | |
Misinjection of tumor cells | Improve injection technique. | |
Poor fluorescent signal of tumor cells | Low expression of fluorophore | Increase ectopic fluorophore expression by re-transducing with lentiviral vector, re-selecting the cells that are high in fluorescence using FACS sorting, or choosing a different promoter to drive stronger expression of the fluorophore. |
Fluorophore is poorly excitable using multiphoton microscopy | Not every fluorophore is easy to excite by multiphoton microscopy. This also depends on the dynamic range of the laser. Literature studies (Drobizhev et al., 2011) and a priori in vitro testing are advised. | |
Background signal in liver | For excitations at ∼800 nm, there is a lot of background fluorescence visible in the liver due to nicotinamide adenine dinucleotide (NADH; Wang et al., 2015) | Choose a different fluorophore to label cells, or optimize the excitation of the fluorophore with different wavelengths. |
Movement of tissue during imaging using AIW | Glue is old and did not result in proper fixation of the liver |
Replace your glue. Be sure to store cyanoacrylate glue at 4°C. Remove the window under anesthesia, so that the animal can still be used for immediate short-term imaging using surgical exposure. |
Premature death of mouse | Constipation due to intestines glued to liver |
Adjust liver and intestines properly before gluing the window. Remove the window under anesthesia, so that the animal can still be used for immediate short-term imaging using surgical exposure. |
Excess blood loss during or after surgery | Make sure all wounds are properly closed after surgery. To promote recovery after blood loss, 50-200 μl sterile PBS can be injected s.c. after surgery. | |
Poor visibility of liver | Exudate formed underneath the coverglass |
To prevent this, make sure to properly glue the window to the liver, making a tight seal and leaving no gap. In case of replaceable window: Remove coverglass, peel away exudate, flush with PBS, and place new clean coverglass. In case of normal window: Sacrifice the animal or remove window under anesthesia and perform surgical exposure for immediate short-term imaging. |
Time Considerations
Cell culture
Depending on the proliferation rates of the cell line, vials of cells should be thawed between 1 and 2 weeks before injection and the cells passaged at least once. Harvesting the cancer cells before injection should take <45 min but can take longer depending on the cell line and the number of cells used for injection. Cells should be kept on ice until injection, which should be ≤1 hr later. If this takes >1 hr, harvest cells for a single injection and repeat for additional injections.
Mesenteric vein injection
The preparation of the surgery will take ∼30 min. In experienced hands, the mesenteric vein surgery and injection can be accomplished in 30 min. Bleeding after the injection should stop within a few minutes. The recovery time of the animals after the mesenteric vein injection without abdominal imaging window implantation should be 5-10 min when isoflurane is used as anesthesia. Due to the timeline of harvesting and injection of the tumor cells, it is advisable not to perform more than 10 surgeries per day.
Implantation of abdominal imaging window
Preparation of the liver prior to the implantation can be time consuming and will determine the successful implantation of the window, as incorrect fixation may result in more exudate formation, more tissue movement, and the inability to visualize the liver altogether. In experienced hands, this will take ∼15-30 min. After applying glue, the window should be placed on the liver within 30 s. After window placement, hold pressure on the window for 1 min, and thereafter leave the window untouched for ≥5 min to solidify the glue. During this time, the suture can be placed. After suturing the window in the skin, place the animal in a prone position for 5-10 min to allow the liver to settle. The recovery of the mice from mesenteric vein injection followed by the abdominal imaging window should take <10 min.
Intravital imaging by surgical exposure
Correct fixation of the liver will determine the success of the imaging, as incorrect fixation results in too much tissue movement, which hampers imaging. Therefore, it can be time consuming, but should not take >1 hr. The liver can be imaged by surgical exposure <8 hr. To study migration, 10-min intervals are suited for single positions. For tile scans, 45 min to 1 hr are suitable.
Intravital imaging using abdominal imaging window
For repeated intravital imaging, the time of each individual imaging session should be kept to a minimum. Preparing the animal before imaging and locating the tumor cells will take between 15 and 30 min. When imaged daily, keep imaging sessions <2 hr, and give animals 24-hr breaks between sessions. Recovery of mice from anesthesia after an imaging session should occur within 5 min. Depending on the state of the animal, it is advisable not to image more than 5 days in 2 weeks. When longer timepoints are wanted, animals can be imaged every other day, or less frequently, to allow better recovery. The last timepoint can last longer, as the mouse will be sacrificed after that imaging session.
Acknowledgments
Studies in our laboratory on cancer cell imaging are supported by Cancer Genomics Centre Netherlands (CGC.NL) to LR and PtD. This work is part of the research program 016.176.081 (Veni) to LR, which is financed by the Dutch Organization for Scientific Research (NWO). The authors would also like to thank the LUMC Gisela Their Fellowship and the Leids Universiteits Fonds (LUF) for grant support to LR (CWB 7204). The authors thank the CCB light microscopy facility and Willem Sloos for their support, the LUMC PDC for animal support, and LUMC Facility Management for creating the custom-made microscopy insert and imaging windows.
Author Contributions
Dieuwke L. Marvin: Conceptualization, Data curation, Investigation, Methodology, Writing-original draft, Peter ten Dijke: Funding acquisition, Supervision, Writing-review & editing, Laila Ritsma: Conceptualization, Funding acquisition, Supervision, Writing-review & editing.
Conflict of Interest Statement
The authors declare no potential conflict of interest.
Open Research
Data Availability Statement
Data sharing not applicable to this article as no datasets were generated or analysed during the current study.
Literature Cited
- Allen, T. M., Brehm, M. A., Bridges, S., Ferguson, S., Kumar, P., Mirochnitchenko, O., … PrabhuDas, M. (2019). Humanized immune system mouse models: Progress, challenges and opportunities. Nature Immunology , 20(7), 770–774. doi: 10.1038/s41590-019-0416-z.
- Auguste, P., Fallavollita, L., Wang, N., Burnier, J., Bikfalvi, A., & Brodt, P. (2007). The host inflammatory response promotes liver metastasis by increasing tumor cell arrest and extravasation. The American Journal of Pathology , 170(5), 1781–1792. doi: 10.2353/ajpath.2007.060886.
- Beerling, E., Ritsma, L., Vrisekoop, N., Derksen, P. W. B., & Van Rheenen, J. (2011). Intravital microscopy: New insights into metastasis of tumors. Journal of Cell Science , 124(Pt 3), 299–310. doi: 10.1242/jcs.072728.
- Ben-David, U., Siranosian, B., Ha, G., Tang, H., Oren, Y., Hinohara, K., … Golub, T. R. (2018). Genetic and transcriptional evolution alters cancer cell line drug response. Nature , 560(7718), 325–330. doi: 10.1038/s41586-018-0409-3.
- Brighton, H. E., Angus, S. P., Bo, T., Roques, J., Tagliatela, A. C., Darr, D. B., … Bear, J. E. (2018). New mechanisms of resistance to MEK inhibitors in melanoma revealed by intravital imaging. Cancer Research , 78(2), 542–557. doi: 10.1158/0008-5472.CAN-17-1653.
- Brodt, P. (2016). Role of the microenvironment in liver metastasis: From pre- to prometastatic niches. Clinical Cancer Research , 22(24), 5971–5982. doi: 10.1158/1078-0432.CCR-16-0460.
- Brown, E., McKee, T., DiTomaso, E., Pluen, A., Seed, B., Boucher, Y., & Jain, R. K. (2003). Dynamic imaging of collagen and its modulation in tumors in vivo using second-harmonic generation. Nature Medicine , 9(6), 796–800. doi: 10.1038/nm879.
- Chen, N., Ritsma, L. M. A., & Vrisekoop, N. (2019). In vivo characteristics of human and mouse breast tumor cell lines. Experimental Cell Research , 381(1), 86–93. doi: 10.1016/j.yexcr.2019.04.009.
- Clark, A. M., Ma, B., Taylor, D. L., Griffith, L., & Wells, A. (2016). Liver metastases: Microenvironments and ex-vivo models. Experimental Biology and Medicine , 241(15), 1639–1652. doi: 10.1177/1535370216658144.
- Drobizhev, M., Makarov, N. S., Tillo, S. E., Hughes, T. E., & Rebane, A. (2011). Two-photon absorption properties of fluorescent proteins. Nature Methods , 8(5), 393–399. doi: 10.1038/nmeth.1596.
- Fumagalli, A., Oost, K. C., Kester, L., Morgner, J., Bornes, L., Bruens, L., … van Rheenen, J. (2020). Plasticity of Lgr5-negative cancer cells drives metastasis in colorectal cancer. Stem Cell , 26, 569–578.e7. doi: 10.1016/j.stem.2020.02.008.
- Giampieri, S., Pinner, S., & Sahai, E. (2010). Intravital imaging illuminates transforming growth factor β signaling switches during metastasis. Cancer Research , 70(9), 3435–3439. doi: 10.1158/0008-5472.CAN-10-0466.
- Gligorijevic, B., Kedrin, D., Segall, J. E., Condeelis, J., & van Rheenen, J. (2009). Dendra2 photoswitching through the mammary imaging window. Journal of Visualized Experiments , 28(June), e1278. doi: 10.3791/1278.
- Goddard, E. T., Fischer, J., & Schedin, P. (2016). A portal vein injection model to study liver metastasis of breast cancer. Journal of Visualized Experiments , 2016(118), 1–10. doi: 10.3791/54903.
- Gomez-Cuadrado, L., Tracey, N., Ma, R., Qian, B., & Brunton, V. G. (2017). Mouse models of metastasis: Progress and prospects. DMM Disease Models and Mechanisms , 10(9), 1061–1074. doi: 10.1242/dmm.030403.
- Gül, N., Babes, L., Siegmund, K., Korthouwer, R., Bögels, M., Braster, R., … Van Egmond, M. (2014). Macrophages eliminate circulating tumor cells after monoclonal antibody therapy. Journal of Clinical Investigation , 124(2), 812–823. doi: 10.1172/JCI66776.
- Headley, M. B., Bins, A., Nip, A., Roberts, E. W., Looney, M. R., Gerard, A., & Krummel, M. F. (2016). Visualization of immediate immune responses to pioneer metastatic cells in the lung. Nature , 531(7595), 513–517. doi: 10.1038/nature16985.
- Hess, K. R., Varadhachary, G. R., Taylor, S. H., Wei, W., Raber, M. N., Lenzi, R., & Abbruzzese, J. L. (2006). Metastatic patterns in adenocarcinoma. Cancer , 106(7), 1624–1633. doi: 10.1002/cncr.21778.
- Higashijima, J., Shimada, M., Chikakiyo, M., Miyatani, T., Yoshikawa, K., Nishioka, M., … Kurita, N. (2009). Effect of splenectomy on antitumor immune system. Anticancer Research , 29(1), 385–393. Retrieved from https://pubmed.ncbi.nlm.nih.gov/19331177/.
- Hirata, E., Girotti, M. R., Viros, A., Hooper, S., Spencer-Dene, B., Matsuda, M., … Sahai, E. (2015). Intravital imaging reveals how BRAF inhibition generates drug-tolerant microenvironments with high integrin Β1/FAK signaling. Cancer Cell , 27(4), 574–588. doi: 10.1016/j.ccell.2015.03.008.
- Huey, D. D., & Niewiesk, S. (2018). Production of humanized mice through stem cell transfer. Current Protocols in Mouse Biology , 8(1), 17–27. doi: 10.1002/cpmo.38.
- Karagiannis, G. S., Pastoriza, J. M., Wang, Y., Harney, A. S., Entenberg, D., Pignatelli, J., … Oktay, M. H. (2017). Neoadjuvant chemotherapy induces breast cancer metastasis through a TMEM-mediated mechanism. Science Translational Medicine , 9(397), eaan0026. doi: 10.1126/scitranslmed.aan0026.
- Kudur, M. H., Pai, S. B., Sripathi, H., & Prabhu, S. (2009). Sutures and suturing techniques in skin closure. Indian Journal of Dermatology, Venereology and Leprology , 75(4), 425. doi: 10.4103/0378-6323.53155.
- Kuo, T.-H.-H., Kubota, T., Nishibori, H., Watanabe, M., Furukawa, T., Kase, S., … Kitajima, M. (1993). Experimental cancer chemotherapy using a liver metastatic model of human colon cancer transplanted into the spleen of severe combined immunodeficient mice. Journal of Surgical Oncology , 52(2), 92–96. doi: 10.1002/jso.2930520207.
- Lamb, R., Ozsvari, B., Lisanti, C. L., Tanowitz, H. B., Howell, A., Martinez-Outschoorn, U. E., … Lisanti, M. P. (2015). Antibiotics that target mitochondria effectively eradicate cancer stem cells, across multiple tumor types: Treating cancer like an infectious disease. Oncotarget , 6(7), 4569–4584. doi: 10.18632/oncotarget.3174.
- Landreau, P., Drouillard, A., Launoy, G., Ortega-Deballon, P., Jooste, V., Lepage, C., … Bouvier, A.-M. (2015). Incidence and survival in late liver metastases of colorectal cancer. Journal of Gastroenterology and Hepatology , 30(1), 82–85. doi: 10.1111/jgh.12685.
- Liu, Y., Mi, Y., Mueller, T., Kreibich, S., Williams, E. G., Van Drogen, A., … Aebersold, R. (2019). Multi-omic measurements of heterogeneity in HeLa cells across laboratories. Nature Biotechnology , 37(3), 314–322. doi: 10.1038/s41587-019-0037-y.
- Luzzi, K. J., MacDonald, I. C., Schmidt, E. E., Kerkvliet, N., Morris, V. L., Chambers, A. F., & Groom, A. C. (1998). Multistep nature of metastatic inefficiency: Dormancy of solitary cells after successful extravasation and limited survival of early micrometastases. American Journal of Pathology , 153(3), 865–873. doi: 10.1016/S0002-9440(10)65628-3.
- Margarido, A. S., Bornes, L., Vennin, C., & van Rheenen, J. (2019). Cellular plasticity during metastasis: New insights provided by intravital microscopy. Cold Spring Harbor Perspectives in Medicine , 10(11), a037267. doi: 10.1101/cshperspect.a037267.
- Marvin, D. L., Heijboer, R., ten Dijke, P., & Ritsma, L. (2020). TGF-β signaling in liver metastasis. Clinical and Translational Medicine , 10(7), e160. doi: 10.1002/ctm2.160.
- Matsuda, M., & Terai, K. (2020). Experimental pathology by intravital microscopy and genetically encoded fluorescent biosensors. Pathology International , 70(7), 12925. doi: 10.1111/pin.12925.
- Muta, Y., Fujita, Y., Sumiyama, K., Sakurai, A., Taketo, M. M., Chiba, T., … Imajo, M. (2018). Composite regulation of ERK activity dynamics underlying tumour-specific traits in the intestine. Nature Communications , 9(1), 1–16. doi: 10.1038/s41467-018-04527-8.
- Overwijk, W. W., & Restifo, N. P. (2000). B16 as a mouse model for human melanoma. Current Protocols in Immunology , 39(1), 20.1.1–20.1.29. doi: 10.1002/0471142735.im2001s39.
- Poste, G., Doll, J., Hart, I. R., & Fidler, I. J. (1980). In vitro selection of murine B16 melanoma variants with enhanced tissue-invasive properties. Cancer Research , 40(5), 1636–1644.
- Pritchett-Corning, K. R., Mulder, G. B., Luo, Y., & White, W. J. (2010). Principles of rodent surgery for the new surgeon. Journal of Visualized Experiments , 47(January), e2586. doi: 10.3791/2586.
- Ritsma, L., Steller, E. J. A., Beerling, E., Loomans, C. J. M., Zomer, A., Gerlach, C., … van Rheenen, J. (2012). Intravital microscopy through an abdominal imaging window reveals a pre-micrometastasis stage during liver metastasis. Science Translational Medicine , 4(158), 158ra145–158ra145. doi: 10.1126/scitranslmed.3004394.
- Schindelin, J., Arganda-Carreras, I., & Frise, E. et al. (2012). Fiji: an open-source platform for biological-image analysis. Nature Methods , 9(7), 676–682. doi: 10.1038/nmeth.2019.
- Ritsma, L., Steller, E. J. A., Ellenbroek, S. I. J., Kranenburg, O., Rinkes, I. H. M.B., & Van Rheenen, J. (2013). Surgical implantation of an abdominal imaging window for intravital microscopy. Nature Protocols , 8(3), 583–594. doi: 10.1038/nprot.2013.026.
- Soares, K. C., Foley, K., Olino, K., Leubner, A., Mayo, S. C., Jain, A., … Zheng, L. (2014). A preclinical murine model of hepatic metastases. Journal of Visualized Experiments , 91(September), 51677. doi: 10.3791/51677.
- Speak, A. O., Swiatkowska, A., Karp, N. A., Arends, M. J., Adams, D. J., & Van Der Weyden, L. (2017). A high-throughput in vivo screening method in the mouse for identifying regulators of metastatic colonization. Nature Protocols , 12(12), 2465–2477. doi: 10.1038/nprot.2017.118.
- Stacey, G. N. (2011). Cell culture contamination. Methods in Molecular Biology , 731, 79–91. doi: 10.1007/978-1-61779-080-5_7.
- Steeg, P. S. 2016. Targeting metastasis. Nature Reviews Cancer. 16(4): 201–218. doi: 10.1038/nrc.2016.25.
- Te Velde, E. A., Wagenaar, G. T. M., Reijerkerk, A., Roose-Girma, M., Borel Rinkes, I. H. M., Voest, E. E., … Meijers, J. C. M. (2003). Impaired healing of cutaneous wounds and colonic anastomoses in mice lacking thrombin-activatable fibrinolysis inhibitor. Journal of Thrombosis and Haemostasis , 1(10), 2087–2096. doi: 10.1046/j.1538-7836.2003.00404.x.
- Van Der Bij, G. J., Bögels, M., Otten, M. A., Oosterling, S. J., Kuppen, P. J., Meijer, S., … Van Egmond, M. (2010). Experimentally induced liver metastases from colorectal cancer can be prevented by mononuclear phagocyte-mediated monoclonal antibody therapy. Journal of Hepatology , 53(4), 677–685. doi: 10.1016/j.jhep.2010.04.023.
- Vidal-Vanaclocha, F. (2008). The prometastatic microenvironment of the liver. Cancer Microenvironment , 1(1), 113–129. doi: 10.1007/s12307-008-0011-6.
- Vidal-Vanaclocha, F. (2011). Architectural and functional aspects of the liver with implications for cancer metastasis. Cancer Metastasis - Biology and Treatment , 16(1), 9–42. doi: 10.1007/978-94-007-0292-9_2.
- Wang, H., Liang, X., Mohammed, Y. H., Thomas, J. A., Bridle, K. R., Thorling, C. A., … Roberts, M. S. (2015). Real-time histology in liver disease using multiphoton microscopy with fluorescence lifetime imaging. Biomedical Optics Express , 6(3), 780. doi: 10.1364/boe.6.000780.
- Weil, S., Osswald, M., Solecki, G., Grosch, J., Jung, E., Lemke, D., … Winkler, F. (2017). Tumor microtubes convey resistance to surgical lesions and chemotherapy in gliomas. Neuro-Oncology , 19(10), 1316–1326. doi: 10.1093/neuonc/nox070.
- Welch, D. R., & Hurst, D. R. (2019). Defining the hallmarks of metastasis. Cancer Research , 79(12), 3011–3027, doi: 10.1158/0008-5472.CAN-19-0458.
- Yamasaki, M., Takemasa, I., Komori, T., Watanabe, S., Sekimoto, M., Doki, Y., … Monden, M. (2007). The gene expression profile represents the molecular nature of liver metastasis in colorectal cancer. International Journal of Oncology , 30(1), 129–138. doi: 10.3892/ijo.30.1.129.
- Zhang, G., & Du, Y. C. N. (2019). Orthotopic pancreatic tumor mouse models of liver metastasis. Methods in Molecular Biology , 1882, 309–320. doi: 10.1007/978-1-4939-8879-2_27.
Citing Literature
Number of times cited according to CrossRef: 3
- Dieuwke L. Marvin, Jelmer Dijkstra, Rabia M. Zulfiqar, Michiel Vermeulen, Peter ten Dijke, Laila Ritsma, TGF-β Type I Receptor Signaling in Melanoma Liver Metastases Increases Metastatic Outgrowth, International Journal of Molecular Sciences, 10.3390/ijms24108676, 24 , 10, (8676), (2023).
- Jorge Almagro, Hendrik A. Messal, Volume imaging to interrogate cancer cell-tumor microenvironment interactions in space and time, Frontiers in Immunology, 10.3389/fimmu.2023.1176594, 14 , (2023).
- Idaira M. Guerrero Fonseca, Michael Schnoor, Eduardo Vadillo, Intravital microscopy, Cell Movement in Health and Disease, 10.1016/B978-0-323-90195-6.00009-7, (323-339), (2022).