Genome Editing with CRISPR-Cas: An Overview
G. Brett Robb, G. Brett Robb
Abstract
The understanding and application of clustered regularly interspaced short palindromic repeat (CRISPR) systems and CRISPR-associated (Cas) nucleases have helped genome editing become a standard laboratory technique. CRISPR-Cas nucleases are RNA-programmed DNA-cutting enzymes that facilitate the introduction of intentional sequence changes into the genomes of experimental cells and organisms. This overview provides a background for genome editing and CRISPR-Cas nucleases, as well as an overview of the technology used to assess the outcomes of genome editing. © 2019 The Authors.
INTRODUCTION
Probably no more high-profile technology has been developed in the biological sciences within the last decade than CRISPR-Cas nuclease–based genome editing. This is with good reason. The application of easily programmable CRISPR-Cas nucleases to the already active field of genome editing has helped it take leaps forward and has made genome editing of model (and not traditionally model) organisms accessible to many more laboratories. The applications of CRISPR-Cas technology to genome editing are many. We have seen the technology significantly advance our understanding of gene and genome structure and function, make previously inaccessible model organisms genetically tractable, and become applied to drug development and therapy.
The purpose of this overview is to set the stage for understanding CRISPR-Cas nuclease protocols. Basic concepts and the process of genome editing are discussed. We further highlight the components required to perform CRISPR-Cas genome editing, where to get them, and things to think about pre- and post-experiment.
THE BASICS OF GENOME EDITING
To begin, we should make some distinctions between the terms “genome engineering,” “genome editing,” and “gene editing.” Genome engineering is the process of making designed modifications to the sequence of genomic DNA. Gene editing and genome editing are techniques for genome engineering that involve DNA repair mechanisms for incorporating site-specific modifications into genomic DNA. Gene editing is distinguished from genome editing by focusing only on genes. Genome editing is the preferred term for the subject of this article, since many genome-engineering efforts aim to make targeted changes to non-gene regions in order to insert new genes or to modify gene-regulatory regions so as to manipulate the functions of existing genes.
Why Would You Want to Edit a Genome?
Reasons to edit a genome are manifold. For research, genome editing enables classic loss- and gain-of-function experiments to be performed to elucidate gene contributions to phenotypes.
Functional gene knockouts can be produced by disrupting the open reading frames of protein-coding regions, or by larger-scale deletions. Similarly, the function of regulatory elements such as promoters, enhancers, and microRNA response elements can be disrupted using CRISPR-Cas targeting approaches.
When combined with readily available synthetic or recombinant DNA, CRISPR-Cas genome editing allows for the relatively easy insertion of exogenous sequences including reporter genes, genome-regulatory elements, and genetic circuits into experimental organisms. Furthermore, in high-value strains of an organism: e.g., crop plants, genes conferring desirable traits can be “stacked” at particular loci for ease of subsequent propagation.
Highlights from the History of Genome Editing
Genome editing is not a new field and has in fact been an active one for decades. The critical developments leading to the current state of the field have been reviewed in-depth, and the reader is referred to excellent reviews by Carroll (2014) and Urnov (2018).
The key background points for the purposes of this overview are the following: first, genome editing as performed today relies on inherent cellular mechanisms of genomic DNA repair using either homology-dependent repair or end-joining pathways; second, double-stranded DNA breaks (DSBs) greatly increase the likelihood of incorporating a nucleic acid at or near the break site; third, and tying the first two points together, targeted nucleases that induce DNA breaks at desired regions direct DNA repair mechanisms to areas of interest for the purpose of producing an edit (Fig. 1).
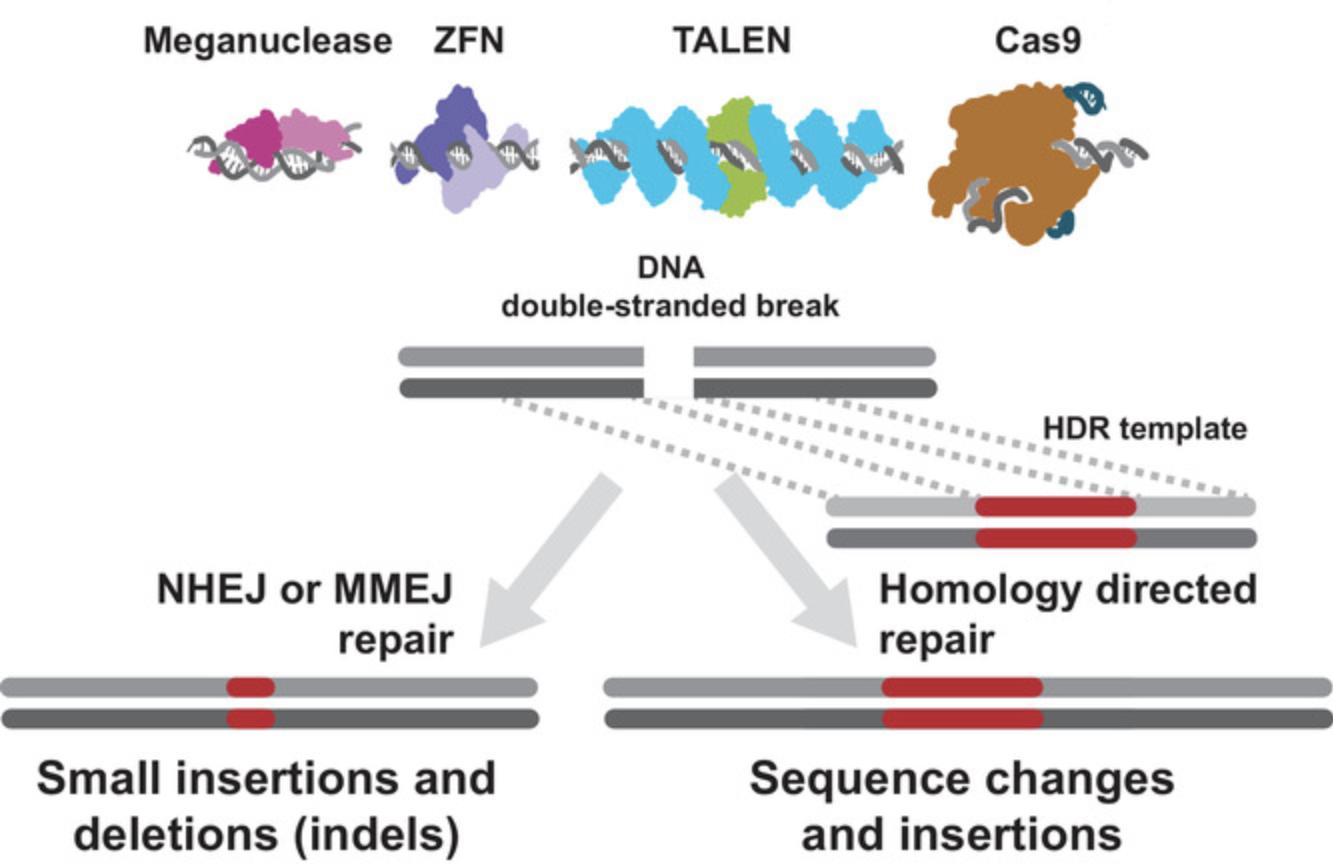
The earliest genome-editing work in fact did not capitalize on induced dsDNA breaks at all, instead relying on long regions of homology intended to direct an exogenous DNA sequence to a particular region of the genome (reviewed in Urnov, 2018; see “34-18 B.C.”).
A major advance was achieved when the Jasin lab showed that ectopic expression of a meganuclease (I-Sce I, a long-recognition-sequence endonuclease) of yeast origin in mouse embryonic stem cells caused double-stranded DNA breaks that were repaired by non-homologous end joining or by homology-directed repair using an exogenous DNA template (Rouet, Smih, & Jasin, 1994). The rate of homology-directed repair strikingly increased when a DSB was introduced, as compared to the rate without the DSB. DSBs repaired without a template contained a variety of small deletion mutations, some indicative of repair based on regions or microhomology (fewer than 10 bases). This group of discoveries set the stage for the nuclease-assisted genome-editing developments that followed.
The Carroll laboratory, building upon this discovery, advanced the concept of targeting DSB-inducing endonucleases in order to create genome edits at any desired location. To do so, they used zinc-finger nucleases (ZFN). ZFN are engineered nucleases made up of zinc-finger arrays designed to bind to specific DNA sequences fused to DNA endonuclease domains. Working in Drosophila , Carroll and collaborators showed that expression of a ZFN targeting the yellow gene produced 6% mutant phenotypes—a high rate for the time—and that the yellow gene in the mutant animals contained small insertions and deletions at the target of the nuclease (Bibikova, Golic, Golic, & Carroll, 2002). Soon after, the Carroll group, and separately, the Baltimore lab reported the introduction of specific mutations into genomic loci using ZFN and exogenous repair templates in Drosophila (Bibikova, Beumer, Trautman, & Carroll, 2003) and cultured human cells (Porteus & Baltimore, 2003), respectively. Shortly thereafter, a group from Sangamo BioSciences demonstrated the use of this approach to edit a clinically relevant locus in clinically relevant cells (Urnov et al., 2005), showing that the approach can be ported from model systems to systems that were previously inaccessible.
In the years that immediately followed, further advances were achieved in the realm of new targeted nucleases and their application to model and non-model organisms. In particular, TAL effector nucleases (TALEN) and methods for rapidly generating them brought another approach to targeting DNA DSBs for genome engineering (reviewed in Carroll, 2014; Urnov, 2018). TALENs are conceptually similar to ZFN and meganucleases in that the proteins must be re-engineered for each target DNA sequence (Joung & Sander, 2013). While useful for cases where extensive optimization is acceptable (e.g., clinical use, biotech crop development), this requirement for protein engineering limited the versatility of nuclease-assisted genome editing, especially in the research setting. The discovery and characterization of CRISPR-Cas systems and their implementation significantly moved genome editing from the realm of a relatively small community of specialists toward broad application as a research tool.
CRISPR-Cas
The discovery of CRISPR-Cas systems pre-dated the Jasin lab's fundamental discoveries about dsDNA break repair. Originally observed as an interesting feature in Escherichia coli genome sequences (Ishino, Shinagawa, Makino, Amemura, & Nakata, 1987; Nakata, Amemura, & Makino, 1989), several years of work from a number of groups in academia and industry went on to establish that CRISPR-Cas systems function as adaptive immune systems in bacteria and archaea, protecting them from invading viral or plasmid nucleic acids (reviewed in Doudna & Charpentier, 2014).
Biochemical characterization and informatic analyses of CRISPR-Cas systems revealed their striking diversity and widespread distribution (Koonin, Makarova, & Zhang, 2017). Many thousands of CRISPR-Cas systems have been identified, and they are currently divided into six different types based on the signature Cas proteins, their sequence similarity, and the organization of Cas genes. The Cas proteins are confusingly named. For example, Cas1 is an enzyme involved in the adaptation phase of CRISPR-Cas-mediated immunity, while the group of effector nucleases that cut invading DNA includes Cas9 and Cas12, which are routinely used in genome editing. The group of effector nucleases also includes Cas3, Cas8, Cas10, and Cas13, which are not routinely used (reviewed in Makarova, Wolf, & Koonin, 2018).
In general, CRISPR-Cas systems use a genome-encoded guide RNA to recruit nuclease activities to DNA or RNA in a sequence-specific manner. Of particular relevance to genome editing, a small subset of CRISPR systems use a single protein that facilitates both the targeting activity conferred by guide-RNA binding and double-stranded DNA endonuclease activity. The recognition of these activities in CRISPR-Cas9 and its subsequent adaptation for programmable targeting of genomic DNA in non-bacterial cells were the major advances that enabled genome editing to be applied as broadly as it is today. The reason for this is that Cas9 and Cas12a allow for changing the target of the nuclease by simply changing the guide RNA—a process requiring a few days at most. This contrasts with the much more laborious re-engineering or selection of meganuclease, ZFN, or TALEN proteins for each new genome target, which requires weeks to months or more.
How Do Cas Nucleases Work?
The CRISPR-Cas systems routinely used for genome editing today are the Type II Cas9 and Type V Cas12a systems, though some recent reports have used examples of the more complicated multi-component type I systems (Hidalgo-Cantabrana, Goh, Pan, Sanozky-Dawes, & Barrangou, 2019; also see Fig. 2). Only Cas9 and Cas12a are discussed further.
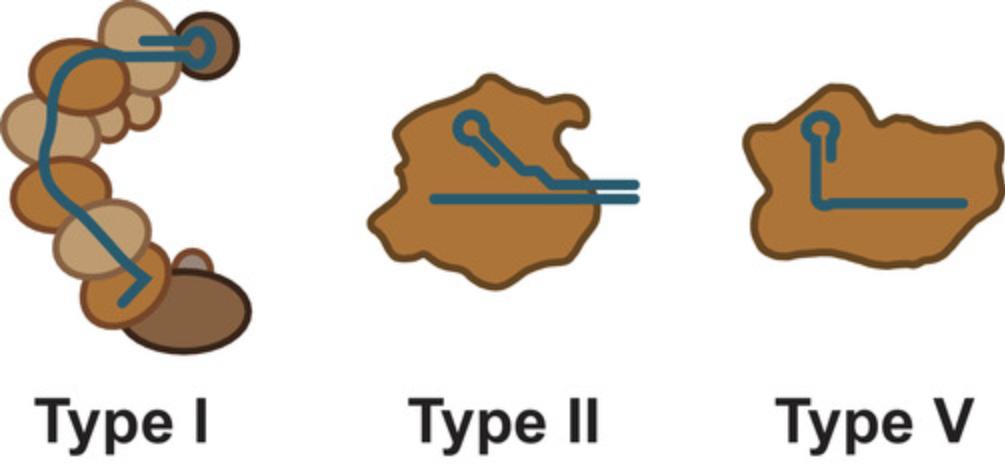
Guide RNAs
Cas9 and Cas12a are both single-protein endonucleases that interact with RNAs guiding them to a DNA sequence they will cut. For Cas9, the natural guide RNAs are composed of two parts—a CRISPR RNA (crRNA) and a trans -activating CRISPR RNA (tracrRNA; reviewed in Charpentier, Richter, van der Oost, & White, 2015; Fig. 3). The crRNA part of the gRNA is derived from a long transcript encoded in the CRISPR array that contains one or more spacer-repeat units. In this context, the spacer encodes the targeting specificity of the crRNA, and the repeat is required for Cas9 binding. The sequences contained in the spacers represent bacterial immune memory and are derived from the DNA sequence of previously encountered invasive DNA. In a mature crRNA for Cas9, the target-specific region is ∼20 bases in length.
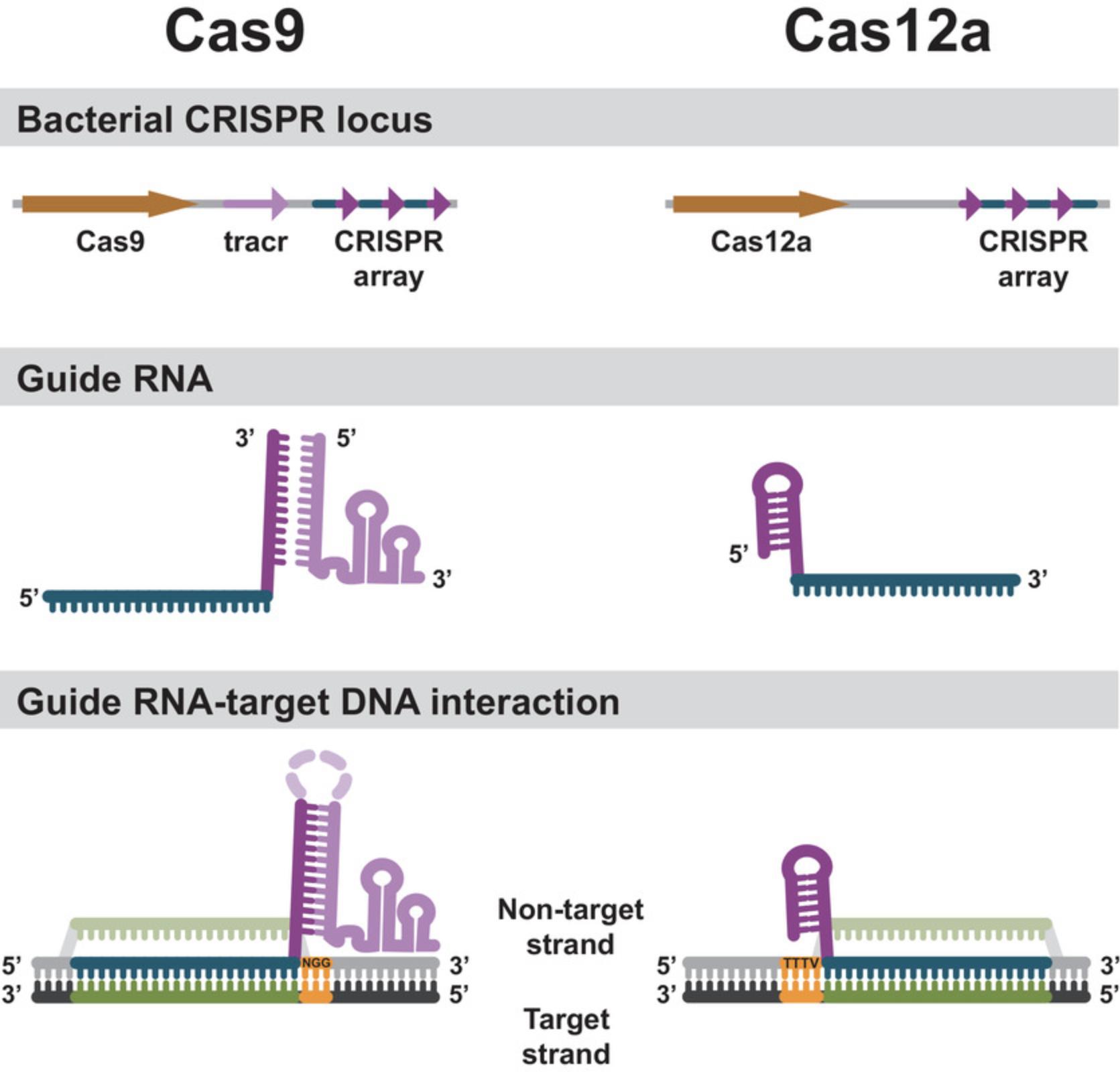
The other component of the functional Cas9 guide is derived from a tracrRNA transcript that is typically encoded near the CRISPR array. The crRNA and the tracrRNA contain a region of mutual complementarity from the repeat unit of the CRISPR array and an anti-repeat region in the tracrRNA transcript. The interaction of the repeat and anti-repeat forms an extended RNA duplex. In bacterial cells, this duplex is shortened by an intracellular ribonuclease to ∼24 bp (Deltcheva et al., 2011). For many Cas9 applications, the crRNA and tracrRNA can be joined in this duplex region to produce a single guide RNA (sgRNA) of ∼100 bases in length (Jinek et al., 2012; Fig. 3).
CRISPR-Cas12a systems use a simpler variation of this scheme, without tracrRNA. The CRISPR arrays in Cas12a systems are transcribed as long transcripts containing repeats and spacers and subsequently processed by Cas12a itself into a repeat-spacer unit–containing guide RNA (Fig. 2). These are simply called crRNAs or gRNAs (Zetsche et al., 2015). The target-specific region in a mature gRNA for Cas12a is ∼20 bases in length.
For both Cas9 and Cas12a, the gRNA contains a region that is specific to the Cas nuclease. This makes Cas9 and Cas12a distinct from one another while being similar in function and mechanism. The protein-specific RNA region interacts with the Cas nuclease to form a ribonucleoprotein complex (RNP) in which the protein has an altered conformation when compared to the protein without guide RNA. The conformational change positions the target-specific region of the gRNA in the complex in a configuration primed for strand annealing, forming an active DNA surveillance complex (Sternberg, Redding, Jinek, Greene, & Doudna, 2014; Swarts, van der Oost, & Jinek, 2017) (Fig. 4).
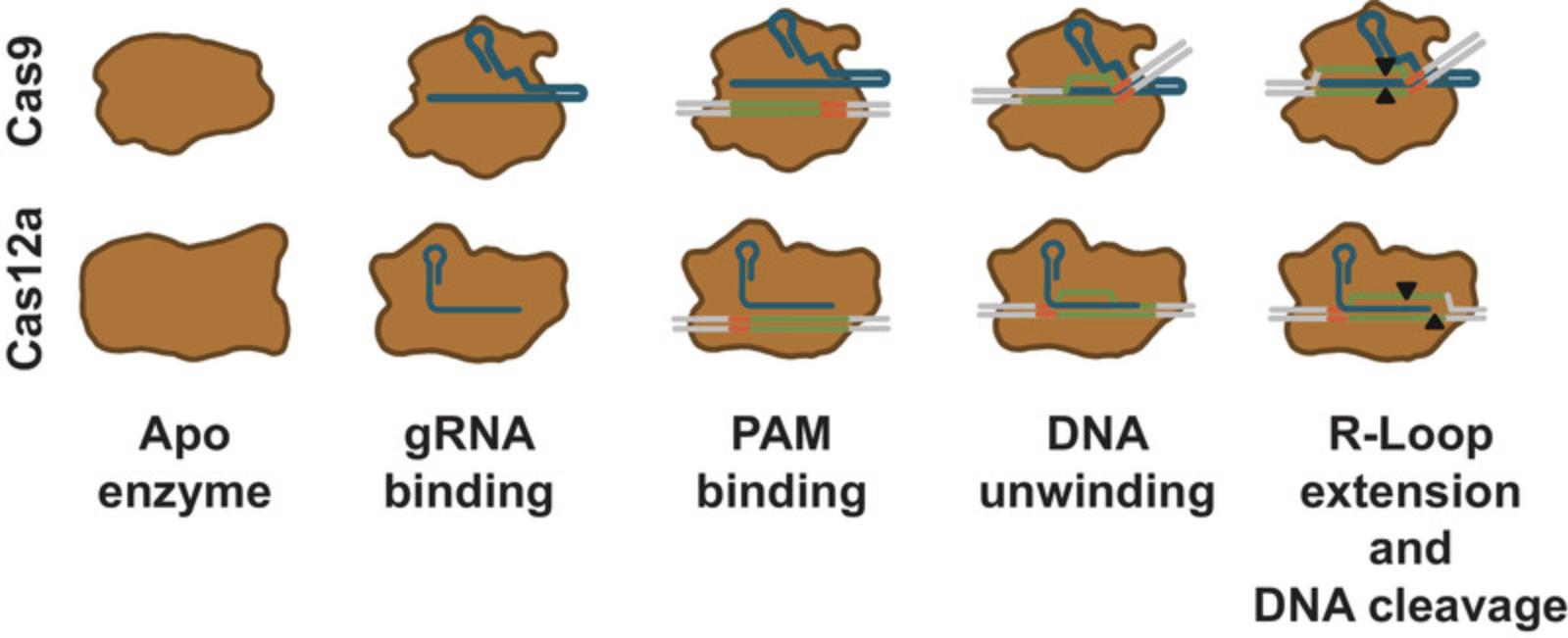
DNA target search
The Cas nuclease RNP complex requires base pairing between the target-specific region of the guide RNA and the target DNA. In addition, a conserved sequence adjacent to the target region, called the protospacer adjacent motif (PAM) sequence, must be present (Gasiunas, Barrangou, Horvath, & Siksnys, 2012; Jinek et al., 2012; Zetsche et al., 2015). The PAM sequence is recognized by the nuclease itself and is not base-paired to the guide RNA (Anders, Niewoehner, Duerst, & Jinek, 2014; Gao, Yang, Rajashankar, Huang, & Patel, 2016; Nishimasu et al., 2014; Yamano et al., 2017). The sequence of the PAM is critical for Cas nuclease function. For the commonly used SpyCas9, the PAM sequence is 5′-NGG-3′. For Asp and LbaCas12a, the PAM sequences are TTTV (Zetsche et al., 2015; Fig. 3).
For Cas9, the RNP searches DNA for the correct PAM sequence before base pairing with the guide RNA (Sternberg et al., 2014), and it is likely that Cas12a functions in a similar manner (Swarts et al., 2017). When the Cas nuclease finds its required PAM sequence, the RNP induces local dsDNA melting adjacent to the PAM. Guide RNA strand invasion forms a DNA-RNA duplex that progresses in the direction from the PAM toward the end of the guide RNA target-specific sequence (Sternberg et al., 2014; Szczelkun et al., 2014). The resulting DNA-guide RNA duplex structure with the displaced DNA strand is called the R-loop (Fig. 4).
DNA cleavage
After a Cas nuclease has recognized a PAM and formed an R-loop, it is activated for cleavage (Sternberg et al., 2014). Cas9 has two distinct nuclease domains—an HNH domain and a split RuvC domain. The RuvC domain cuts the DNA backbone on the same strand where the PAM is denoted (i.e., the displaced strand or non-target strand), while the HNH domain cleaves the DNA backbone of the strand that is hybridized to the guide RNA (i.e., the non-PAM strand, or target strand; Gasiunas et al., 2012; Jinek et al., 2012). For SpyCas9, cleavage takes place 3 bp from the NGG PAM, producing mostly blunt-ended DNA fragments (Gasiunas et al., 2012; Jinek et al., 2012). By mutating catalytic residues of the Cas9 nuclease domains, Cas9 nickases have been created that nick on one or the other strand only (Jinek et al., 2012). These so-called nickases can be used in closely spaced pairs targeting opposite strands to achieve genome editing via dsDNA breaks (Ran et al., 2013).
In contrast to Cas9, Cas12a has a single split RuvC nuclease domain (Zetsche et al., 2015). This domain catalyzes the cleavage of the DNA on both strands (Swarts et al., 2017), leaving a staggered end by cutting ∼18 bp from the PAM on the non-target strand and ∼24 bp on the target strand (Zetsche et al., 2015; Fig. 4).
Cas Nucleases Applied to Genome Editing
For purposes of editing a genome, Cas9 or Cas12a is used to target and cleave DNA in the genome of a living cell. Of the two, Cas9 is the more widely used system. Differing PAM requirements may influence an investigator's choice of which system to use.
In its simplest implementation, a guided Cas nuclease produces a single DNA DSB at its genomic target. The genomic target contains the ∼20 base sequence programmed by the guide RNA adjacent to the PAM sequence. This DSB is recognized by the cellular machinery and repaired.
In the absence of a repair template containing homologous sequences at or near the DSB, the repair is mediated by non-homologous end-joining (NHEJ) or microhomology-mediated end joining (MMEJ) pathways. After repair, the NHEJ and MMEJ pathways produce small insertions or deletions (indels). In practice, small indels can disrupt gene-regulatory elements or can result in frameshift mutations in the protein-coding sequences of genes. These outcomes can disrupt the function of the targeted gene or regulatory element. Using a pair of Cas nucleases with spaced targets, it is also possible to induce large deletions or genomic rearrangements, such as inversions or translocations (Chen et al., 2014; Han et al., 2014; and reviewed in Brunet & Jasin, 2018).
DSB may also be repaired using an investigator-supplied template, in which case homology-directed repair (HDR) pathways are used. This approach can be used to change the sequence at or near a genomic target (Fig. 1). For example, HDR can be used to mimic a clinically significant allele of a gene or to change an amino acid within a protein. This approach may also be used to insert relatively large stretches of DNA into a particular genomic location. Examples may include the insertion of a detectable reporter gene such as GFP near a regulatory element, fusion or tagging of a protein of interest with an epitope tag or fluorescent reporter, or the insertion of whole genes or genetic circuits. For both correction and insertion, repair templates are designed to include regions of homologous DNA at their termini that correspond to genomic regions flanking the Cas nuclease–targeted site.
DNA repair pathways differ in various organisms and cell types. Differences will impact the design of repair templates for insertion or correction approaches. Some studies have determined optimal HDR donor configurations for specific applications in specific models systems (see for instance Richardson, Ray, DeWitt, Curie, & Corn, 2016).
The differences in the activities of the DNA repair pathways will also influence the efficiency of producing indel mutations via NHEJ or MMEJ, and even the viability of the targeted cells. Organism-specific protocols will usually contain design considerations for repair templates and expected outcomes of DSB repair.
Paired nickases
Using paired Cas9 nickase mutants is an approach to further increase the specificity of genome editing using Cas9. This strategy usually uses the D10A mutant of SpyCas9, which is deficient in cleaving the non-target strand and thus produces a nick on the target strand. Designing a pair of Cas9 gRNAs for D10A nickases such that a nick is made on one strand of the DNA duplex by one Cas9 nickase RNP while the other member of the pair makes a nick on the other strand functionally produces a DSB and effectively doubles the sequence recognition requirement to do so (Mali, Aach, et al., 2013; Ran et al., 2013). In human cell lines, the preferred configuration is to use SpyCas9 D10A with guide RNAs designed such that the two PAMs are situated outside the nicking sites, and that the nicking sites are 40 to 70 bp apart. SpyCas9 D10A nickases can also be useful for creating insertion-type genome edits using exogenously supplied repair templates (Yan, Schubert, Young, & Wang, 2017).
Base editing
Base editing uses Cas9 D10A nickases fused to engineered base deaminase enzymes to make single-base changes in the DNA sequence without making DNA DSBs (Komor, Kim, Packer, Zuris, & Liu, 2016; Fig. 5). The Cas9 nickase part of the base-editor protein has two functions. The first is to target the deaminase activity to the desired region. The deaminase domains in base editors come in two varieties—adenosine deaminase or cytosine deaminase. The targeted activity of cytosine deaminase changes a C·G base pair to a T·A base pair by converting cytosine to uracil on the non-target strand within the R-loop opened by the Cas9 nickase. The target strand is nicked by the still-functional HNH domain of Cas9 nickase—the second function of the enzyme. DNA nicking stimulates DNA mismatch repair using the deaminated (uracil-containing) strand as a template. The U·A basepair is subsequently converted to T·A via DNA replication or repair.
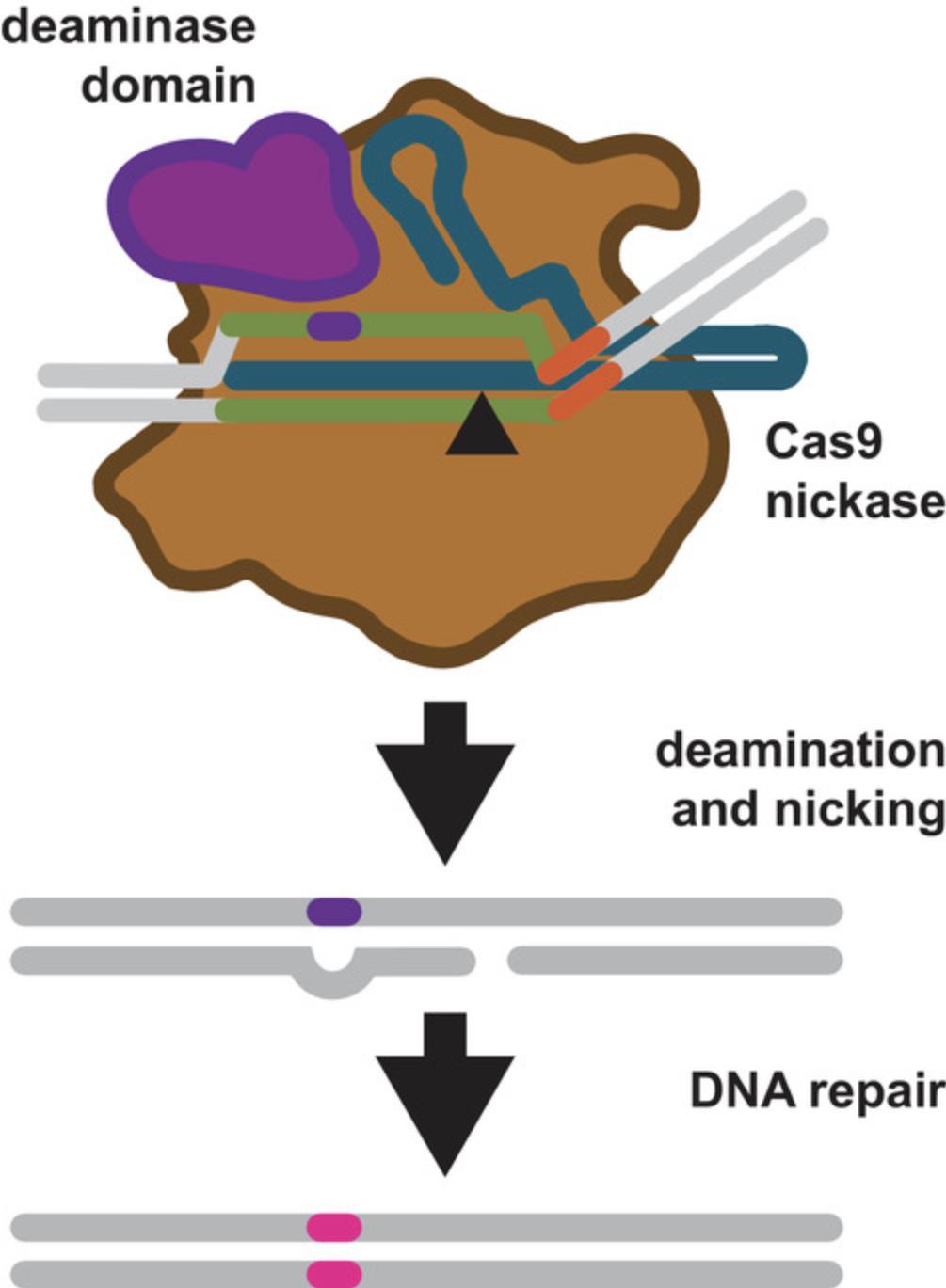
The targeted activity of adenosine deaminase can cause an A·T to G·C sequence change in much the same way. Adenosine is deaminated to inosine, which is subsequently used to repair the nicked strand with a cytosine, and the I·C base pair is resolved to G·C (Gaudelli et al., 2017).
For both cytosine and adenosine base editors, editing can only take place within a defined “window” dictated by Cas9 nickase R-loop formation and the position of the deaminase domain of the fusion protein within the RNP-DNA complex (Gaudelli et al., 2017; Kim et al., 2017; Fig. 5; Komor et al., 2016, 2017). Base-editing windows can complicate the design of experiments, but several gRNA design tools (see below) are now able to aid gRNA design for targeted base editing. Base editors are also currently being revised and improved at a rapid rate, and may become more flexible as future versions are engineered.
Why would one use a base editor instead of HDR? Base editing does not require a DSB, which can result in indel mutations and, rarely, chromosome rearrangements. Base editing does not require an exogenous repair template either (in base editing the strand containing the deaminated base is the repair template, see Fig. 5). Taken together, these factors increase the efficiency of making single-base changes with base editors (Gaudelli et al., 2017; Komor et al., 2016). The caveat is that choice of base-editable sites as targets may be constrained by base-editing windows when compared to targets that can be cleaved by Cas9 nuclease and potentially repaired using HDR to make a single-base change.
CRISPRi, CRISPRa, and other dCas9 applications
CRISPR interference (CRISPRi) and CRISPR activation (CRISPRa) are methods for inhibiting or activating the expression of a gene by targeting genomic regulatory elements (Gilbert et al., 2013; Mali, Esvelt, & Church, 2013; Qi et al., 2013). Technically, these are not genome-editing methods, since no change to the genome is made. However, they can be useful approaches to temporarily turn on/off genes of interest in order to study their function or be useful as custom regulatory components in genetic circuits. Both CRISPRi and CRISPRa rely on cleavage-deficient Cas nucleases, most often S. pyogenes dCas9, and sequence-specifically target regulatory factors to locations on the genome. Often, dCas9 is fused to either a transcription activator or inhibitor domain directly. There are numerous variations on CRISPRi and CRISPRa in terms of regulatory domain fusions, including epigenetic DNA modifiers. In some applications, regulatory domains are recruited to genomic DNA by tethering them to the gRNA of the dCas9 (Zalatan et al., 2015).
Other applications for dCas9 include fusion or tethering of fluorescent proteins or other molecules in order to localize these to a genomic region of interest. Because of the versatility and ease with which Cas nucleases can be programmed, applications for dCas nuclease–based localization of molecules to genomes is limited only by the imagination.
WHAT DO I NEED TO EDIT A GENOME USING CRISPR-Cas NUCLEASES
In theory, the requirements for CRISPR-Cas genome editing are few. They are:
- A Cas nuclease
- A guide RNA
- A template for HDR-based repair (optional)
In practice, there are many ways to generate an active Cas nuclease complex inside a cell. To direct its activity, targets must be picked and gRNAs designed.
Picking Targets and Designing Guide RNAs
Several CRISPR-Cas nuclease gRNA design tools are freely available on the web. Most tools are updated regularly to incorporate their prediction models for on- and off-target activity based on the latest research. Off-target activity includes DNA cleavage at an unintended site that results in a mutation. A short list of target-selection and gRNA-design tools can be found in Table 1. The tools vary in their level of integration with other DNA sequence analysis tools, and also with interfaces that enable the easy ordering of custom gRNA synthesis services.
Tool | URL | Notes |
---|---|---|
Benchling | https://benchling.com | A cloud-based informatics platform with DNA sequence management and integrated CRISPR-Cas nuclease target picking |
Broad Institute GPP sgRNA designer | https://portals.broadinstitute.org/gpp/public/analysis-tools/sgrna-design | Web tool that picks and ranks candidate sgRNA sequences for DNA targets provided |
ChopChop | https://chopchop.cbu.uib.no/ | Web tool for selecting target sites for Cas9, Cas12a, or TALEN-directed mutagenesis |
Horizon/Dharmacon CRISPR Design Tool | https://dharmacon.horizondiscovery.com/gene-editing/crispr-cas9/crispr-design-tool/ | Commercial web tool for selecting targets for Cas nuclease genome editing |
Both on-target and off-target activity prediction models are constantly being improved, and are not perfect. Thus, designing multiple (three to five) gRNAs with different sequences that target the same genomic region of interest is highly recommended. Activity prediction models incorporated in CRISPR-Cas target-picking tools are also diversifying based on application. For instance, some tools have features to simultaneously design oligonucleotide HDR donors, or have specialized searches for CRISPRa or for base-editing applications in addition to CRISPR-Cas knockout applications.
DNA Delivery of Cas Nuclease Editing Components
The Cas nuclease components may be expressed from plasmid DNA. Many organism-specific plasmids for expressing Cas nucleases and their guide RNA scaffolds are available (see Table 2, Where to Obtain CRISPR Genome Editing Reagents). These variations will have the Cas nucleases codon-optimized for translation in the organism of choice and will have promoters appropriate to ensure the transcription of both the mRNA encoding the Cas nuclease and the guide RNA. For each new target, the plasmid containing the gRNA template will need to be modified. This can be rapidly done using modern recombinant DNA manipulation techniques including PCR, mutagenesis, and DNA assembly (see, for example, Hsieh, 2015; Kabadi, Ousterout, Hilton, & Gersbach, 2014; Port, Chen, Lee, & Bullock, 2014; Yin et al., 2015). Addgene is an excellent resource for obtaining plasmid DNA for genome-editing experiments. There are several other commercial sources for Cas nuclease and guide RNA expression plasmids (Table 2).
Source | Notes | |
---|---|---|
Plasmid DNA | ||
Addgene |
|
|
Commercial vendors |
|
|
Chemically synthesized guide RNAs | ||
Eurofins |
|
|
Genscript |
|
|
Horizon|Dharmacon |
|
|
IDT |
|
|
MilliporeSigma |
|
|
Synthego |
|
|
ThermoFisher |
|
|
TriLink Biotechnologies |
|
|
mRNA | ||
ThermoFisher |
|
|
TriLink Biotechnologies |
|
|
Cas nuclease protein | ||
IDT |
|
|
MilliporeSigma |
|
|
NEB |
|
|
Genscript |
|
|
Aldevron |
|
|
Toolgen |
|
|
ThermoFisher |
|
|
ssDNA HDR templates | ||
IDT |
|
|
Genscript |
|
|
Genewiz |
|
|
Takara |
|
|
dsDNA HDR templates | ||
Commercial gene synthesis service providers |
|
Plasmid DNA can be delivered into cells using lipofection, electroporation, or microinjection. The choice of delivery method will be constrained by the feasibility and efficiency of each within a particular model organism. Of note, when Cas nucleases are expressed from DNA in eukaryotic cells, it may be important or even necessary for the Cas nuclease to be fused to nuclear localization signals (NLS). NLS are short peptide sequences that direct proteins translated in the cytoplasm to be imported into the nucleus.
In some experimental systems, it is desirable to deliver CRISPR-Cas genome-editing reagents using viruses. Constructs for viral delivery are also widely available in the community. Changing the target involves the same approaches as for plasmid delivery, e.g., PCR, mutagenesis, and DNA assembly techniques. Overall, a viral delivery approach is more complex than plasmid delivery since viral particles must be generated and purified. However, depending on the experimental system, the viral delivery of CRISPR-Cas reagent can be much more efficient than plasmid transfection or electroporation.
RNA Delivery of Cas Nuclease Editing Reagents
Both the Cas nuclease and the gRNAs can be delivered as RNA molecules. In this scheme, an mRNA encoding a Cas nuclease must be obtained or made in the laboratory. For this approach, it is critical that the mRNA contain organism-appropriate regulatory sequences to ensure the efficient translation of the protein. Like delivery of plasmid-encoded Cas nucleases, it may be important or critical that the encoded proteins be fused to NLS.
For the delivery of gRNAs, there are two general approaches. An investigator can create or obtain transcription templates to direct the in vitro synthesis of the gRNAs. Most often, gRNAs are transcribed in vitro using T7 RNA polymerase, then purified and concentrated. gRNA plasmid templates are widely available and require changing of the target-specific sequences using PCR, mutagenesis, or assembly techniques before use. Alternatively, gRNAs can be chemically synthesized. gRNAs are readily available from a number of service providers including Horizon/Dharmacon and IDT (see Table 2). The monetary cost of gRNAs is significant but dropping, and may be balanced out by savings in labor costs to generate gRNA transcripts in the lab.
Delivery of mRNA is an efficient way to introduce Cas nuclease into cells and organisms. DNA transcription is not required, and higher levels of genome editing have been observed in some systems.
For the co-delivery of Cas nuclease mRNA and gRNA, lipofection, electroporation/nucleofection, or microinjection can be used. An important consideration is the stability of the gRNAs over the time interval required for the Cas nuclease to be translated into protein. To address this, several synthetic oligonucleotide providers have made available gRNAs with stabilizing chemical modifications that likely prolong their intracellular half-lives.
Stabilization of Cas nuclease gRNAs may also be useful in scenarios where Cas nuclease protein is expressed from DNA. gRNAs may be introduced together with a DNA-based expression construct, or independently when Cas nucleases are already expressed in the target cell.
Ribonucleoprotein Complex Delivery of Cas Nuclease Editing Reagents
An alternative approach to delivering the reagents required for CRISPR-Cas genome editing is to assemble gRNAs and Cas nuclease proteins into active RNPs in tubes, and then introduce these directly into cells. Like DNA or RNA, RNPs can be delivered using lipofection, electroporation, or microinjection.
Cas nuclease proteins are commercially available from many reagent companies (see below), or alternatively can be expressed in E. coli and purified in any laboratory equipped to do so. gRNAs can either be produced in the laboratory by in vitro transcription or obtained from service providers that chemically synthesize them.
Delivery of RNP has the advantage of high editing rate, fewer off-target effects, and no chance of inadvertently integrating plasmid DNA into the targeted genome (Kim, Kim, Cho, Kim, & Kim, 2014; Liang et al., 2015; Schumann et al., 2015). In addition, the RNP approach has the advantage of being a single platform that works across species, because organism-specific regulatory sequences are not required to direct transcription of Cas nuclease mRNA or gRNA or to direct translation of the Cas nuclease mRNA. A further advantage is that multiplex experiments are simplified as compared to plasmid and RNA delivery approaches. RNPs programmed separately in vitro can be combined before their introduction into cells.
Repair Templates
The requirements for repair templates will vary by model organism and application (e.g., transgene insertion versus small base changes). For virtually all genome-editing applications, repair templates are DNA. The length of homologous DNA regions, as well as single versus double-stranded DNA, are variations that may be important to consider. A few studies have undertaken a systematic approach for defining what makes an efficient repair template (Richardson et al., 2016; Yan et al., 2017).
Double-stranded DNA templates can be generated using standard recombinant DNA approaches. Usually, these are constructed in plasmids containing inserts designed to have homology to the DNA flanking the target and that contain cargo for insertion or that has been designed to induce other sequence change. Plasmids are often used to make HDR templates because they are easy to manipulate in the laboratory, and it is easy to amplify them in E. coli with extremely high fidelity. Techniques to capture, modify and otherwise manipulate HDR templates in plasmids include PCR, DNA assembly technologies, restriction digestion and ligation, and Golden Gate cloning, as well as site-directed mutagenesis. In addition to plasmids, HDR templates can also be delivered as cargo in viral vectors. The techniques to manipulate the HDR templates are largely the same as for plasmids, but the viral approach adds the complication of production and purification of viral particles.
For HDR, chemically synthesized DNA oligonucleotides/assemblies are an alternative to cloned DNA in plasmids. Some but not all model systems are amenable to this approach, and it is especially useful for introducing small-scale sequence changes. DNA oligonucleotides/assemblies are available as dsDNA (e.g., gBlocks) up to 2 to 3 kbp in length or as chemically synthesized ssDNA oligos up to 500 nt. ssDNA up to ∼5000 nt can also be made from dsDNA, either as a service or using commercially available kits. Note that dsDNA fragments can be amplified in the laboratory using standard PCR, while ssDNA cannot be easily amplified.
HOW TO READ AND SCORE EDITING OUTCOMES
After having delivered CRISPR-Cas reagents into cells, how does one know if editing has occurred? This is an important question to consider as an investigator is designing a genome-editing experiment. It is critical to consider controls that are appropriate for how editing outcomes will be measured.
Phenotype
For some experiments, editing will result in an easily scorable phenotype such as a color change (tyrosinase, yellow), differential sensitivity for a selection agent in the case of gene disruption, or the appearance of a reporter activity such as GFP, or other new function or trait imparted by the insertion of a new gene or change. However, in many cases, the consequences of a genome edit may be more subtle. In any case, the outcomes of genome-editing experiments can be relatively easily determined at the DNA level.
Assessing Genome-Editing Outcomes at the DNA Level
For most approaches to assessing mutations, DNA must be released from the experimental cells and is sometimes purified. Mammalian cultured cells, for example, can simply be lysed in their culture vessels before PCR amplification of targeted regions of the genome. Blood can be used directly as a template with specialized PCR amplification reagent formulations. After release, DNA from genome-edited cells can be amplified and sequenced or analyzed using nuclease digestion. Droplet digital PCR is also used. Protocols for analysis of editing outcomes at the DNA level will differ based on the model organism, but some general principles are applicable to most if not all.
Editing outcomes in individuals or in populations
In some experimental systems, an in-depth analysis of a few to tens of genomes may be warranted. For example, if embryos are being edited in an experiment, genomes from animals may be analyzed using tissue samples from individuals. In this case, an investigator might be interested in asking: How many of the live animals are mutants at the target site? Are the animals heterozygous? Are the animals compound heterozygotes with two different mutant alleles? In the case of a transgene or other larger insertion, an investigator may be interested in characterizing the flanking regions of the insertion carefully—at nucleotide resolution—to examine if open reading frames have been preserved and if any of the targeted loci have been repaired by NHEJ or MMEJ instead of HDR. These might result in indel mutations instead of the desired insertions. In these cases, the sequencing of PCR amplicons generated using primer sets flanking the targeted site, and PCR amplicons generated using primers that amplify the margins of the desired insertion, may be desirable. Critical controls in this scenario are DNA from wild-type (WT) littermates or parental tissue.
In other experimental systems, cultured cells, for example, CRISPR-Cas genome-editing reagents, are introduced into a population of thousands to millions of cells. Some fraction of the cells will receive the editing reagents, and in a fraction of the cells that receive the reagents, they will function as intended, producing an edit. In some scenarios, selective pressure can be applied to enrich the population of edited cells (e.g., antibiotic resistance), but in many experiments, there is no opportunity to apply selective pressure. In this case, an investigator may additionally be interested in asking: What percent of the population of cells has been edited? What mutation variants have been introduced at the targeted locus in the population?
End-joining repair–mediated edits (see above) often produce small indel mutations. These and other small (less than ∼50 bp) sequence changes induced by HDR or other means will usually not impact PCR amplification of genomes containing mixtures of mutant and wild-type DNA using primers flanking the target site. Important controls here might include unperturbed pools of cells, cells having had CRISPR-Cas editing reagents introduced with no targets in the genome of interest, and cells having had CRISPR-Cas editing reagents targeted to a “safe-harbor” locus (see the Glossary in APPENDIX 1).
Methods for assessing editing outcomes
Assessing editing outcomes at the DNA level can generally be performed at “low resolution” or “high resolution.” “Low-resolution” assays have the advantages of providing rapid results and using readily accessible materials, equipment, and analysis resources, but do not reveal the individual identities of mutations at nucleotide resolution. “High-resolution” approaches provide much more detail about editing outcomes—usually nucleotide resolution—and are more quantitative than “low-resolution” assays; however, the former approaches are based on high-throughput sequencing. This requires specialized equipment or services, can add significant cost to an experiment, and can require informatics analysis resources that may not be available in every lab. Table 3 provides a list of methods used for examining genome-editing outcomes.
Resolution | Examples | Advantages | Disadvantages |
---|---|---|---|
Low |
|
|
May provide an underestimate of editing |
|
|
Requires editing site to contain a restriction site | |
|
Rapid Uses standard lab equipment |
Requires the assembly of Cas nuclease RNPs | |
Droplet digital PCR | Quantitative | Requires specialized equipment and optimization of assay design | |
ICE or TIDE analysis of Sanger DNA sequencing |
|
Requires access to Sanger DNA sequencing services | |
High | Deep amplicon sequencing | Provides quantitative information on the extent and sequence of genome edits |
|
Low-resolution assays begin with DNA that has been PCR amplified using primers flanking the intended target. PCR products can then be analyzed by nucleases, or by sequencing (see Fig. 6).
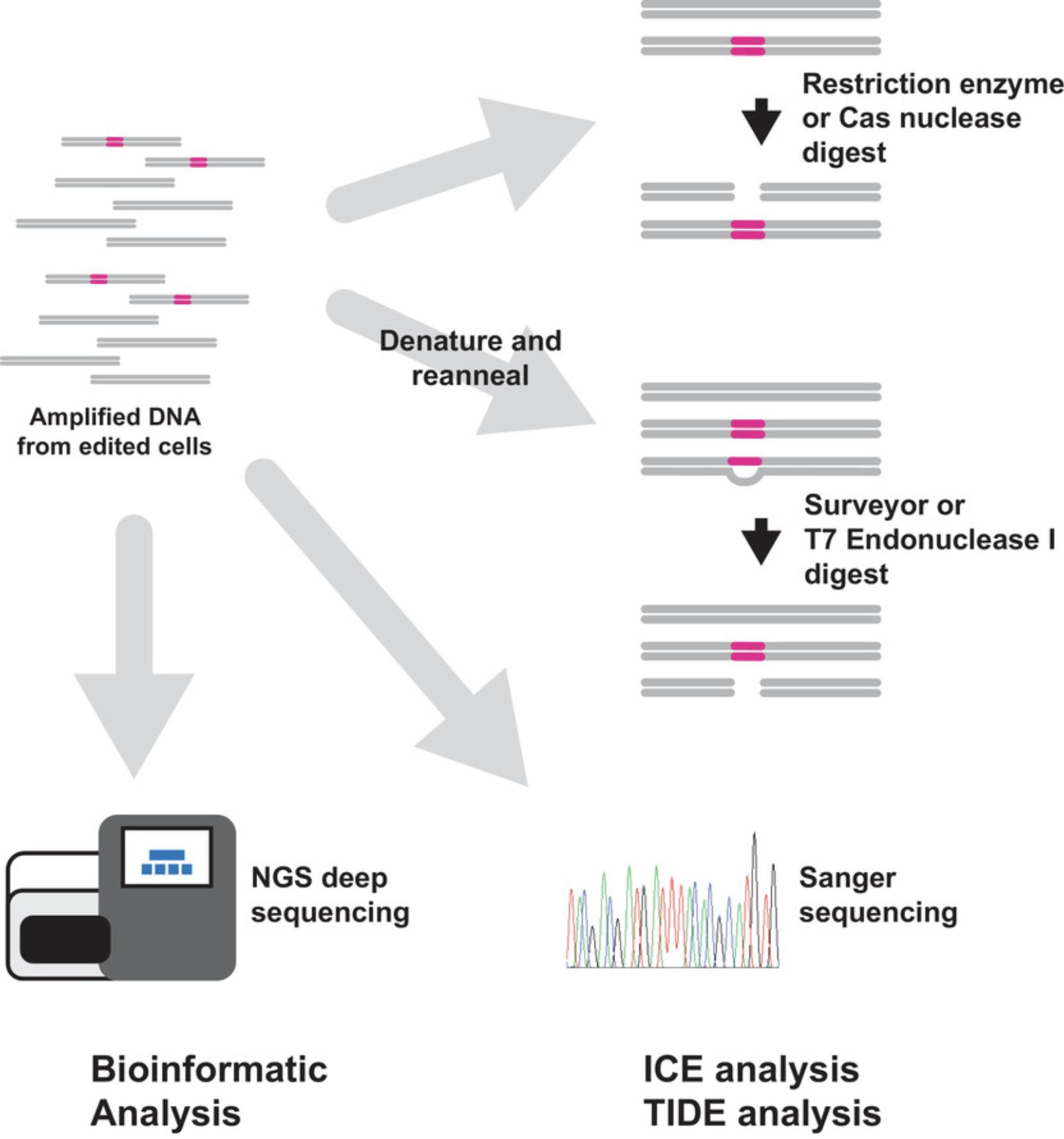
T7 Endonuclease1—a resolvase enzyme that recognizes and cleaves DNA mismatches—or Surveyor (cel1) mismatch endonuclease (Babon, McKenzie, & Cotton, 2003; Qiu et al., 2004; Vouillot, Thelie, & Pollet, 2015)—are used to digest PCR products that have been denatured and reannealed. The reannealing process produces DNA duplexes containing WT and mutant mismatches. These mismatches are cleaved by the endonucleases, and the products are separated by electrophoresis. The degree of editing can be estimated by comparing the amount of intact DNA versus digested DNA. Importantly, perfectly matched DNA will not be digested by either endonuclease. This means that WT DNA will be intact after digestion, but also means that mutant DNAs of the same sequence can reanneal and remain intact, although in cell population analysis (as opposed to analysis of clonal cells, or individuals), this is likely a minor factor considering the wide variety of edited sequence outcomes. Both endonucleases will also cleave DNA with single-base mismatches at a much slower rate than DNA with multiple base mismatches, so this approach may produce an underestimation of the total level of editing in a population and is not appropriate for profiling intentional single-base edits.
An alternative to assessing editing efficiency with mismatch nucleases is to digest the PCR-amplified target site using a nuclease that recognizes the WT DNA sequence. If convenient, an edit can be designed to span a naturally occurring restriction endonuclease site. Any disruption of this site (for instance by an indel) will prevent the restriction endonuclease from cutting the PCR product. Alternatively, an active Cas nuclease RNP can be used to digest the PCR product. Cas nuclease RNPs have robust activity in vitro and have the advantage of being programmable to almost any desired sequence. In addition, if RNP reagents have been introduced to make the intended edit, then the appropriate RNP is already on hand and available to profile editing. Digested PCR products can be resolved by electrophoresis, and in the case of nucleases that recognize the WT unedited sequence, the amount of uncleaved DNA represents mutant DNA, while cleaved fragments contain unedited WT sequence.
An approach that provides some additional detail is bulk Sanger sequencing of PCR products amplified from edited genomic DNA using PCR primers that flank the edited site. In this approach, electronic .abi files containing fluorescent cycle-sequencing results from control and edited samples are uploaded to web servers that analyze and compare the electropherograms to deconvolute editing outcomes. Results provided include the overall extent of editing, some characterization of the indel mutations detected, and their relative proportions (Brinkman, Chen, Amendola, & van Steensel, 2014). Of note, this approach only works for small-scale mutations. Large-scale deletions and transgene insertions may be missed by this approach. There are currently two websites that host this type of analysis—Synthego ICE Analysis, and Desktop Genetics’ TIDE analysis. Both provide graphical results and commentary, as well as troubleshooting guides. Of note, especially to non-academic investigators, is that Desktop Genetics TIDE analysis has a restrictive and aggressively enforced license. Synthego's ICE tool is available to all for free.
“High-resolution” analysis of genome-editing outcomes is based on deep sequencing of PCR amplicons containing the editing target using NGS. These approaches require access to high-throughput sequencing services, which are available at many organizations. In general, high-resolution data analysis will provide details of the extent of editing as well as the frequency and nucleotide identity of mutant alleles.
Sequencing libraries are constructed from edited DNA. This involves adding the appropriate DNA adapters to the ends of the DNA to enable sequencing on the high-throughput sequencing instrument. Most often, this is performed by the investigator using commercially available kits that provide the necessary DNA oligonucleotides, enzymes, and reagents. Sequencing is usually performed by a core facility or service provider, and raw sequence data is returned to the investigator. Typically, thousands to millions of sequencing reads are returned. Analysis of the data requires access to desktop computing resources and some know-how for managing and manipulating large datasets.
There are several widely available informatics tools with web interfaces available for analyzing high-throughput sequencing data. As tools are rapidly changing over time, this overview will not provide an exhaustive list of currently available tools. However, two current tools are highlighted. Galaxy (Afgan et al., 2018) is a web-based, open-source general tool for large-scale data analysis. Genome-editing-specific analysis workflows for use in Galaxy can be customized or obtained via sharing. Crispresso2 (Clement et al., 2019) is an excellent web-based tool designed specifically for analyzing genome-editing events. Data visualization and commentary on editing events including NHEJ, HDR, and base editing are provided by this tool.
What about off-target editing?
In the realm of CRISPR-Cas genome editing, off-target effects have made headlines. Should one worry about off-target effects or unintended edits? The answer is yes, but it is important to contextualize concerns about off-target edits.
As the first point of context, the Cas nucleases used for genome editing have been extensively studied and are highly specific. The enzymes use multiple checkpoints before cutting, including gRNA recognition, PAM recognition, and gRNA-target hybridization to achieve exquisite specificity. After all, off-target cleavage in the context of the bacterial immune system would cause life-threatening autoimmunity. Thus, in the experimental setting, the cause of off-target editing is not likely to be a nonspecific activity of the Cas nucleases. Rather, the cause is most likely to be the guide RNA. Luckily, many guide-RNA design tools now use algorithms that take into account data from experiments that have analyzed the tolerance of the Cas nuclease for mismatches in the gRNA target R-loop. Many tools will advise against targeting regions with high sequence similarity to other genomic regions, and will also advise against targeting repetitive genomic sequences.
Higher-fidelity variants of SpyCas9 have been developed and are available. Using these will likely not be detrimental to a genome-editing experiment, but as discussed above, the advantages of their use with well-designed gRNA may be small.
The second point of context follows. For the users of this overview and the protocol-containing articles that follow, there will not likely be any life-threatening or even life-altering consequences to off-target or unintended editing events. More likely, if any unintended events actually occur, they might complicate the interpretation of the phenotypic data. For example, an investigator might ask the question: What is the function of gene X in process Y? A concern may be that the change in process Y is not a result of the edited gene X but rather the result of an unintended edit not related to gene X. This concern is managed by carefully controlled experiments. Extending the example above, do genome edits to gene X made with two or more different guide RNA sequences result in the same phenotype related to process Y? If the answer is yes, then the phenotype is less likely to have been caused by an undetected off-target or unintended edit. To add another layer of rigor, an investigator could add back the function of gene X by supplying it exogenously to confirm its role in process Y.
Overall, off-target editing effects should be considered, and can be addressed for most if not all applications with good experimental design, target selection, and sgRNA design.
FOR MORE INFORMATION
In addition to the literature referenced below, there are several excellent sources of information that the readers of this unit might find helpful.
Addgene (https://www.addgene.org/), as mentioned in Table 2, is a fantastic resource for how-to's, more detailed information, and discussion of CRISPR-Cas genome-editing papers.
The Innovative Genomics Institute (https://innovativegenomics.org/) is another excellent source of background information, explainers, and a terrific glossary with fun animations (https://innovativegenomics.org/resources/educational-materials/).
Acknowledgments
Thank you to Jennifer Curcuru, Ryan Fuchs, Meg Mabuchi, Ezra Schildkraut, Breton Hornblower, and Tom Evans for their helpful comments on drafts of this overview. My apologies to the authors of all of the great work in the genome editing and CRISPR-Cas fields whose studies I did not discuss here.
Literature Cited
- Afgan, E., Baker, D., Batut, B., van den Beek, M., Bouvier, D., Cech, M., … Blankenberg, D. (2018). The Galaxy platform for accessible, reproducible and collaborative biomedical analyses: 2018 update. Nucleic Acids Research , 46(W1), W537–W544. doi: 10.1093/nar/gky379.
- Anders, C., Niewoehner, O., Duerst, A., & Jinek, M. (2014). Structural basis of PAM-dependent target DNA recognition by the Cas9 endonuclease. Nature , 513, 569–573. doi: 10.1038/nature13579.
- Babon, J. J., McKenzie, M., & Cotton, R. G. H. (2003). The use of resolvases T4 endonuclease VII and T7 endonuclease I in mutation detection. Molecular Biotechnology , 23, 73–81. doi: 10.1385/MB:23:1:73.
- Bibikova, M., Beumer, K., Trautman, J. K., & Carroll, D. (2003). Enhancing gene targeting with designed zinc finger nucleases. Science , 300, 764. doi: 10.1126/science.1079512.
- Bibikova, M., Golic, M., Golic, K. G., & Carroll, D. (2002). Targeted chromosomal cleavage and mutagenesis in Drosophila using zinc-finger nucleases. Genetics , 161, 1169–1175.
- Brinkman, E. K., Chen, T., Amendola, M., & van Steensel, B. (2014). Easy quantitative assessment of genome editing by sequence trace decomposition. Nucleic Acids Research , 42, e168. doi: 10.1093/nar/gku936.
- Brunet, E., & Jasin, M. (2018). Induction of chromosomal translocations with CRISPR-Cas9 and other nucleases: Understanding the repair mechanisms that give rise to translocations. Advances in Experimental Medicine and Biology , 1044, 15–25. doi: 10.1007/978-981-13-0593-1_2.
- Carroll, D. (2014). Genome engineering with targetable nucleases. Annual Review of Biochemistry , 83, 409–439. doi: 10.1146/annurev-biochem-060713-035418.
- Charpentier, E., Richter, H., van der Oost, J., & White, M. F. (2015). Biogenesis pathways of RNA guides in archaeal and bacterial CRISPR-Cas adaptive immunity. FEMS Microbiology Reviews , 39, 428–441. doi: 10.1093/femsre/fuv023.
- Chen, X., Xu, F., Zhu, C., Ji, J., Zhou, X., Feng, X., & Guang, S. (2014). Dual sgRNA-directed gene knockout using CRISPR/Cas9 technology in Caenorhabditis elegans. Scientific Reports , 4, 7581. doi: 10.1038/srep07581.
- Clement, K., Rees, H., Canver, M. C., Gehrke, J. M., Farouni, R., Hsu, J. Y., … Pinello, L. (2019). CRISPResso2 provides accurate and rapid genome editing sequence analysis. Nature Biotechnology , 37, 224–226. doi: 10.1038/s41587-019-0032-3.
- Deltcheva, E., Chylinski, K., Sharma, C. M., Gonzales, K., Chao, Y., Pirzada, Z. A., … Charpentier, E. (2011). CRISPR RNA maturation by trans-encoded small RNA and host factor RNase III. Nature , 471, 602–607. doi: 10.1038/nature09886.
- Doudna, J. A., & Charpentier, E. (2014). The new frontier of genome engineering with CRISPR-Cas9. Science , 346, 1258096. doi: 10.1126/science.1258096.
- Gao, P., Yang, H., Rajashankar, K. R., Huang, Z., & Patel, D. J. (2016). Type V CRISPR-Cas Cpf1 endonuclease employs a unique mechanism for crRNA-mediated target DNA recognition. Cell Research , 26, 901–913. doi: 10.1038/cr.2016.88.
- Gasiunas, G., Barrangou, R., Horvath, P., & Siksnys, V. (2012). Cas9-crRNA ribonucleoprotein complex mediates specific DNA cleavage for adaptive immunity in bacteria. Proceedings of the National Academy of Sciences of the United States of America , 109(39), E2579–E2586. doi: 10.1073/pnas.1208507109.
- Gaudelli, N. M., Komor, A. C., Rees, H. A., Packer, M. S., Badran, A. H., Bryson, D. I., & Liu, D. R. (2017). Programmable base editing of A•T to G•C in genomic DNA without DNA cleavage. Nature , 551, 464–471. doi: 10.1038/nature24644.
- Gilbert, L. A., Larson, M. H., Morsut, L., Liu, Z., Brar, G. A., & Torres, S. E., … Qi, L. S. (2013). CRISPR-mediated modular RNA-guided regulation of transcription in eukaryotes. Cell , 154, 442–451. doi: 10.1016/j.cell.2013.06.044.
- Han, J., Zhang, J., Chen, L., Shen, B., Zhou, J., Hu, B., … Zhang, W. (2014). Efficient in vivo deletion of a large imprinted lncRNA by CRISPR/Cas9. RNA Biology , 11, 829–835. doi: 10.4161/rna.29624.
- Hidalgo-Cantabrana, C., Goh, Y. J., Pan, M., Sanozky-Dawes, R., & Barrangou, R. (2019). Genome editing using the endogenous type I CRISPR-Cas system in Lactobacillus crispatus. Proceedings of the National Academy of Sciences of the United States of America , 116, 15774–15783. doi: 10.1073/pnas.1905421116.
- Hsieh, P. (2015). Construction of an sgRNA-Cas9 expression vector via single-stranded DNA oligo bridging of double-stranded DNA fragments. New England Biolabs Inc. Retrieved from https://www.neb.com/-/media/catalog/application-notes/construction-of-an-sgrna-cas9-expression-vector-e2621.pdf?rev=1128b9c9bc6c4873822da500532ebbb9.
- Ishino, Y., Shinagawa, H., Makino, K., Amemura, M., & Nakata, A. (1987). Nucleotide sequence of the iap gene, responsible for alkaline phosphatase isozyme conversion in Escherichia coli , and identification of the gene product. Journal of Bacteriology , 169, 5429–5433. doi: 10.1128/jb.169.12.5429-5433.1987.
- Jinek, M., Chylinski, K., Fonfara, I., Hauer, M., Doudna, J. A., & Charpentier, E. (2012). A Programmable dual-RNA−guided DNA endonuclease in adaptive bacterial immunity. Science , 337, 816–821. doi: 10.1126/science.1225829.
- Joung, J. K., & Sander, J. D. (2013). TALENs: A widely applicable technology for targeted genome editing. Nature Reviews Molecular Cell Biology , 14, 49–55. doi: 10.1038/nrm3486.
- Kabadi, A. M., Ousterout, D. G., Hilton, I. B., & Gersbach, C. A. (2014). Multiplex CRISPR/Cas9-based genome engineering from a single lentiviral vector. Nucleic Acids Research , 42, e147. doi: 10.1093/nar/gku749.
- Kim, S., Kim, D., Cho, S. W., Kim, J., & Kim, J. S. (2014). Highly efficient RNA-guided genome editing in human cells via delivery of purified Cas9 ribonucleoproteins. Genome Research , 24, 1012–1019. doi: 10.1101/gr.171322.113.
- Kim, Y. B., Komor, A. C., Levy, J. M., Packer, M. S., Zhao, K. T., & Liu, D. R. (2017). Increasing the genome-targeting scope and precision of base editing with engineered Cas9-cytidine deaminase fusions. Nature Biotechnology , 35, 371–376. doi: 10.1038/nbt.3803.
- Komor, A. C., Kim, Y. B., Packer, M. S., Zuris, J. A., & Liu, D. R. (2016). Programmable editing of a target base in genomic DNA without double-stranded DNA cleavage. Nature , 533, 420–424. doi: 10.1038/nature17946.
- Komor, A. C., Zhao, K. T., Packer, M. S., Gaudelli, N. M., Waterbury, A. L., Koblan, L. W., … Liu, D. R. (2017). Improved base excision repair inhibition and bacteriophage Mu Gam protein yields C:G-to-T: A base editor with higher efficiency and product purity. Science Advances , 3(8), eaao4774. doi: 10.1126/sciadv.aao4774.
- Koonin, E. V., Makarova, K. S., & Zhang, F. (2017). Diversity, classification and evolution of CRISPR-Cas systems. Current Opinion in Microbiology , 37, 67–78. doi: 10.1016/j.mib.2017.05.008.
- Liang, X., Potter, J., Kumar, S., Zou, Y., Quintanilla, R., Sridharan, M., … Chesnut, J. D. (2015). Rapid and highly efficient mammalian cell engineering via Cas9 protein transfection. Journal of Biotechnology , 208, 44–53. doi: 10.1016/j.jbiotec.2015.04.024.
- Makarova, K. S., Wolf, Y. I., & Koonin, E. V. (2018). Classification and nomenclature of CRISPR-Cas systems: Where from here? The CRISPR Journal , 1, 325–336. doi: 10.1089/crispr.2018.0033.
- Mali, P., Aach, J., Stranges, P. B., Esvelt, K. M., Moosburner, M., Kosuri, S., … Church, G. M. (2013). CAS9 transcriptional activators for target specificity screening and paired nickases for cooperative genome engineering. Nature Biotechnology , 31, 833–838. doi: 10.1038/nbt.2675.
- Mali, P., Esvelt, K. M., & Church, G. M. (2013). Cas9 as a versatile tool for engineering biology. Nature Methods , 10, 957–963. doi: 10.1038/nmeth.2649.
- Nakata, A., Amemura, M., & Makino, K. (1989). Unusual nucleotide arrangement with repeated sequences in the Escherichia coli K-12 chromosome. Journal of Bacteriology , 171, 3553–3556. doi: 10.1128/jb.171.6.3553-3556.1989.
- Nishimasu, H., Ran, F. A., Hsu, P. D., Konermann, S., Shehata, S. I., Dohmae, N., … Nureki, O. (2014). Crystal structure of Cas9 in complex with guide RNA and target DNA. Cell , 156, 935–949. doi: 10.1016/j.cell.2014.02.001.
- Porteus, M. H., & Baltimore, D. (2003). Chimeric nucleases stimulate gene targeting in human cells. Science , 300, 763. doi: 10.1126/science.1078395.
- Port, F., Chen, H.-M., Lee, T., & Bullock, S. L. (2014). Optimized CRISPR/Cas tools for efficient germline and somatic genome engineering in Drosophila. Proceedings of the National Academy of Sciences of the United States of America , 111, E2967–E2976. doi: 10.1073/pnas.1405500111.
- Qi, L. S., Larson, M. H., Gilbert, L. A., Doudna, J. A., Weissman, J. S., Arkin, A. P., & Lim, W. A. (2013). Repurposing CRISPR as an RNA-guided platform for sequence-specific control of gene expression. Cell , 152, 1173–1183. doi: 10.1016/j.cell.2013.02.022.
- Qiu, P., Shandilya, H., D'Alessio, J. M., O'Connor, K., Durocher, J., & Gerard, G. F. (2004). Mutation detection using Surveyor nuclease. BioTechniques , 36, 702–707. doi: 10.2144/04364pf01.
- Ran, F. A., Hsu, P. D., Lin, C.-Y., Gootenberg, J. S., Konermann, S., Trevino, A. E., … Zhang, F. (2013). Double nicking by RNA-guided CRISPR Cas9 for enhanced genome editing specificity. Cell , 154, 1380–1389. doi: 10.1016/j.cell.2013.08.021.
- Richardson, C. D., Ray, G. J., DeWitt, M. A., Curie, G. L., & Corn, J. E. (2016). Enhancing homology-directed genome editing by catalytically active and inactive CRISPR-Cas9 using asymmetric donor DNA. Nature Biotechnology , 34, 339–344. doi: 10.1038/nbt.3481.
- Rouet, P., Smih, F., & Jasin, M. (1994). Introduction of double-strand breaks into the genome of mouse cells by expression of a rare-cutting endonuclease. Molecular and Cellular Biology , 14, 8096–8106. doi: 10.1128/mcb.14.12.8096.
- Schumann, K., Lin, S., Boyer, E., Simeonov, D. R., Subramaniam, M., Gate, R. E., … Marson, A. (2015). Generation of knock-in primary human T cells using Cas9 ribonucleoproteins. Proceedings of the National Academy of Sciences of the United States of America , 112, 10437–10442. doi: 10.1073/pnas.1512503112.
- Sternberg, S. H., Redding, S., Jinek, M., Greene, E. C., & Doudna, J. A. (2014). DNA interrogation by the CRISPR RNA-guided endonuclease Cas9. Nature , 507, 62–67. doi: 10.1038/nature13011.
- Swarts, D. C., van der Oost, J., & Jinek, M. (2017). Structural basis for guide RNA processing and seed-dependent DNA targeting by CRISPR-Cas12a. Molecular Cell , 66, 221–233.e4. doi: 10.1016/j.molcel.2017.03.016.
- Szczelkun, M. D., Tikhomirova, M. S., Sinkunas, T., Gasiunas, G., Karvelis, T., Pschera, P., … Seidel, R. (2014). Direct observation of R-loop formation by single RNA-guided Cas9 and Cascade effector complexes. Proceedings of the National Academy of Sciences of the United States of America , 111, 9798–9803. doi: 10.1073/pnas.1402597111.
- Urnov, F. D. (2018). Genome editing B.C. (Before CRISPR): Lasting lessons from the “Old Testament.” The CRISPR Journal , 1, 34–46. doi: 10.1089/crispr.2018.29007.fyu.
- Urnov, F. D., Miller, J. C., Lee, Y.-L., Beausejour, C. M., Rock, J. M., Augustus, S., … Holmes, M. C. (2005). Highly efficient endogenous human gene correction using designed zinc-finger nucleases. Nature , 435, 646–651. doi: 10.1038/nature03556.
- Vouillot, L., Thelie, A., & Pollet, N. (2015). Comparison of T7E1 and surveyor mismatch cleavage assays to detect mutations triggered by engineered nucleases. G3 (Bethesda) , 5, 407–415. doi: 10.1534/g3.114.015834.
- Yamano, T., Zetsche, B., Ishitani, R., Zhang, F., Nishimasu, H., & Nureki, O. (2017). Structural basis for the canonical and non-canonical PAM recognition by CRISPR-Cpf1. Molecular Cell , 67, 633–645.e3. doi: 10.1016/j.molcel.2017.06.035.
- Yan, S., Schubert, M., Young, M., & Wang, B. (2017). Applications of Cas9 nickases for genome engineering (CRS-10084-AN). Integrated DNA Technologies. Retrieved from https://sfvideo.blob.core.windows.net/sitefinity/docs/default-source/application-note/applications-of-cas9-nickases-for-genome-engineering.pdf?sfvrsn=70b03707_16.
- Yin, L., Maddison, L. A., Li, M., Kara, N., LaFave, M. C., Varshney, G. K., … Chen, W. (2015). Multiplex conditional mutagenesis using transgenic expression of Cas9 and sgRNAs. Genetics , 200, 431–441. doi: 10.1534/genetics.115.176917.
- Zalatan, J. G., Lee, M. E., Almeida, R., Gilbert, L. A., Whitehead, E. H., La Russa, M., … Lim, W. A. (2015). Engineering complex synthetic transcriptional programs with CRISPR RNA scaffolds. Cell , 160, 339–350. doi: 10.1016/j.cell.2014.11.052.
- Zetsche, B., Gootenberg, J. S., Abudayyeh, O. O., Slaymaker, I. M., Makarova, K. S., Essletzbichler, P., … Koonin, E. V. (2015). Cpf1 is a single RNA-guided endonuclease of a class 2 CRISPR-Cas system. Cell , 163, 759–771. doi: 10.1016/j.cell.2015.09.038.
Citing Literature
Number of times cited according to CrossRef: 15
- Maryam Taghdiri, Claudio Mussolino, Viral and Non-Viral Systems to Deliver Gene Therapeutics to Clinical Targets, International Journal of Molecular Sciences, 10.3390/ijms25137333, 25 , 13, (7333), (2024).
- Nadia Tavakolidakhrabadi, Francesco Aulicino, Carl J May, Moin A Saleem, Imre Berger, Gavin I Welsh, Genome editing and kidney health, Clinical Kidney Journal, 10.1093/ckj/sfae119, 17 , 5, (2024).
- Avinash Singh, G. Dalton Smedley, Jamee-Grace Rose, Kristina Fredriksen, Ying Zhang, Ling Li, Shauna H. Yuan, A high efficiency precision genome editing method with CRISPR in iPSCs, Scientific Reports, 10.1038/s41598-024-60766-4, 14 , 1, (2024).
- Fasiha Qurashi, Syed Riaz Ahmed, Muhammad Yousaf Shani, Muhammad Sayyam Tariq, Hamda Hussain Qureshi, Improvement of ornamental plants through CRISPR-Cas, CRISPRized Horticulture Crops, 10.1016/B978-0-443-13229-2.00007-7, (291-308), (2024).
- Alyona Sorokina, Elizaveta Anchakova, Erdem Dashinimaev, Strategies for HIV-1 suppression through key genes and cell therapy, Frontiers in Medicine, 10.3389/fmed.2023.1259995, 10 , (2023).
- Megan N. Moran, D. B. Jones, S. A. Jensen, R. Marcoli, D. R. Jerry, Optimising commercial traits through gene editing in aquaculture: Strategies for accelerating genetic improvement, Reviews in Aquaculture, 10.1111/raq.12889, 16 , 3, (1127-1159), (2023).
- Wajid Hussain, Xiaohan Yang, Mati Ullah, Huan Wang, Ayesha Aziz, Fang Xu, Muhammad Asif, Muhammad Wajid Ullah, Shenqi Wang, Genetic engineering of bacteriophages: Key concepts, strategies, and applications, Biotechnology Advances, 10.1016/j.biotechadv.2023.108116, 64 , (108116), (2023).
- Olga I. Kershanskaya, Gulvira L. Yessenbaeva, Darya S. Nelidova, Aizhan N. Karabekova, Zarina N. Sadullaeva, RETRACTED: CRISPR/Cas genome editing perspectives for barley breeding, Physiologia Plantarum, 10.1111/ppl.13686, 174 , 3, (2022).
- Thomas Kocher, Igor Petkovic, Johannes Bischof, Ulrich Koller, Current developments in gene therapy for epidermolysis bullosa, Expert Opinion on Biological Therapy, 10.1080/14712598.2022.2049229, 22 , 9, (1137-1150), (2022).
- Dito Anurogo, Nova Yuli Prasetyo Budi, Mai-Huong Thi Ngo, Yen-Hua Huang, Jeanne Adiwinata Pawitan, Cell and Gene Therapy for Anemia: Hematopoietic Stem Cells and Gene Editing, International Journal of Molecular Sciences, 10.3390/ijms22126275, 22 , 12, (6275), (2021).
- Anuradha Bhardwaj, Vikrant Nain, TALENs—an indispensable tool in the era of CRISPR: a mini review, Journal of Genetic Engineering and Biotechnology, 10.1186/s43141-021-00225-z, 19 , 1, (125), (2021).
- Tobias Wimmer, Dileep Bonthu, Vincent Moeschl, Philipp Kleekamp, Christian Thiel, Lyubomyr Lytvynchuk, Matthew Ellinwood, Knut Stieger, A Bioluminescence Resonance Energy Transfer–Based Reporter System: Characterization and Applications, The CRISPR Journal, 10.1089/crispr.2021.0023, 4 , 6, (884-895), (2021).
- Kevin Bloh, Rohan Kanchana, Pawel Bialk, Kelly Banas, Zugui Zhang, Byung-Chun Yoo, Eric B. Kmiec, Deconvolution of Complex DNA Repair (DECODR): Establishing a Novel Deconvolution Algorithm for Comprehensive Analysis of CRISPR-Edited Sanger Sequencing Data, The CRISPR Journal, 10.1089/crispr.2020.0022, 4 , 1, (120-131), (2021).
- Ahmad M. Khalil, The genome editing revolution: review, Journal of Genetic Engineering and Biotechnology, 10.1186/s43141-020-00078-y, 18 , 1, (68), (2020).
- Peter Kalds, Yawei Gao, Shiwei Zhou, Bei Cai, Xingxu Huang, Xiaolong Wang, Yulin Chen, Redesigning small ruminant genomes with CRISPR toolkit: Overview and perspectives, Theriogenology, 10.1016/j.theriogenology.2020.02.015, 147 , (25-33), (2020).